Journal of Animal Science and Biotechnology
Featured articles.

Probiotic Lactobacillus rhamnosus GG improves insulin sensitivity and offspring survival via modulation of gut microbiota and serum metabolite in a sow model (Tianle Gao et al.)
Advances in single-cell transcriptomics in animal research (Yunan Yan et al.)
Call for papers.
We invite you to submit an article to the following thematic series:
Mucosal immunology for nutritional impacts on young animals Submission deadline: December 31, 2024
- Most accessed
- Collections
Productive and metabolomic consequences of arginine supplementation in sows during different gestation periods in two different seasons
Authors: Sara Virdis, Diana Luise, Federico Correa, Luca Laghi, Norma Arrigoni, Roxana Elena Amarie, Andrea Serra, Giacomo Biagi, Clara Negrini, Francesco Palumbo and Paolo Trevisi
Altered microRNA composition in the uterine lumen fluid in cattle ( Bos taurus ) pregnancies initiated by artificial insemination or transfer of an in vitro produced embryo
Authors: Fernando H. Biase, Sarah E. Moorey, Julie G. Schnuelle, Soren Rodning, Martha Sofia Ortega and Thomas E. Spencer
Effects of replacing soybean meal with enzymolysis-fermentation compound protein feed on growth performance, apparent digestibility of nutrients, carcass traits, and meat quality in growing-finishing pigs
Authors: Yu Cheng, Jun He, Ping Zheng, Jie Yu, Junning Pu, Zhiqing Huang, Xiangbing Mao, Yuheng Luo, Junqiu Luo, Hui Yan, Aimin Wu, Bing Yu and Daiwen Chen
Higher abundance of DLD protein in buffalo bull spermatozoa causes elevated ROS production leading to early sperm capacitation and reduction in fertilizing ability
Authors: Seema Karanwal, Ankit Pal, Fanny Josan, Aditya Patel, Jatinder Singh Chera, Sonam Yadav, Vikrant Gaur, Preeti Verma, Shiva Badrhan, Vitika Chauhan, Mukesh Bhakat, Tirtha Kumar Datta and Rakesh Kumar
Dietary supplementation of valine, isoleucine, and tryptophan may overcome the negative effects of excess leucine in diets for weanling pigs containing corn fermented protein
Authors: Andrea P. Mallea, Charmaine D. Espinosa, Su A Lee, Minoy A. Cristobal, Leidy J. Torrez-Mendoza and Hans H. Stein
Most recent articles RSS
View all articles
Structures and characteristics of carbohydrates in diets fed to pigs: a review
Authors: Diego M. D. L. Navarro, Jerubella J. Abelilla and Hans H. Stein
Lysozyme as an alternative to growth promoting antibiotics in swine production
Authors: W. T. Oliver and J. E. Wells
GMOs in animal agriculture: time to consider both costs and benefits in regulatory evaluations
Authors: Alison L Van Eenennaam
Clostridium species as probiotics: potentials and challenges
Authors: Pingting Guo, Ke Zhang, Xi Ma and Pingli He
Microbial synthesis of zinc oxide nanoparticles and their potential application as an antimicrobial agent and a feed supplement in animal industry: a review
Authors: Hidayat Mohd Yusof, Rosfarizan Mohamad, Uswatun Hasanah Zaidan and Nor’ Aini Abdul Rahman
Most accessed articles RSS
Article collection Pan-genome research in farm animal science
Thematic series Advances in silage research Edited by: Prof. Xusheng Guo Collection published: 22 January 2024
Cross-journal collection Gut microbiome and animal health Collection published: 16 November 2022
Thematic series Poultry genetics and genomics Edited by: Prof. Jie Wen Collection published: 11 October 2022
Thematic series Interplay between feed, additives, and gut health of monogastric animals Edited by: Prof. Rajesh Jha Collection published: 6 October 2022
Thematic series Nutritional modulation of embryonic development and potential in livestock and poultry Edited by: Prof. Xiangfang Zeng Collection published: 2 March 2022
Thematic series Biological feed and animal gut health Edited by: Prof. Haifeng Wang Collection published: 12 October 2021
Thematic series Mycotoxin Control in Animal Feed Edited by: Prof. Lv-Hui Sun Collection published: 11 October 2021
Thematic series The progress of low protein diet in poultry Edited by: Prof. Jianmin Yuan Collection published: 6 January 2021
Thematic series Advances on the interactions among nutrition, genomics, and physiology in dairy cattle Edited by: Prof Juan J. Loor Collection published: 29 June 2020 Special Issue Special Issue for JASB 10th Anniversary Edited by: Prof Dorian Garrick Collection published: 17 April 2020
Thematic series Gut microbiota of poultry Edited by: Prof Guolong Zhang Collection published: 13 March 2020
Thematic series Pig gut microbiota: Challenges and opportunities to improve the pig Edited by: Prof Paolo Trevisi and Prof Jürgen Zentek Collection published: 24 May 2019
Thematic series Frontier research in intestinal health of pigs and chickens Edited by: Prof Sung Woo Kim Collection published: 14 January 2019
Thematic series Genome editing in domestic animals Edited by: Prof Jae Yong Han Collection published: 29 January 2018
Thematic series Gastrointestinal microbial ecology and functionality Edited by: Prof Jianxin Liu, Prof Weiyun Zhu Collection published: 8 November 2016
Thematic series Alternatives to antibiotics as growth promoters for use in swine production Edited by: Prof Phil Thacker Collection published: 21 October 2015
Thematic series Fat nutrition and metabolism in food animals Edited by: Prof Jack Odle Collection published: 21 May 2015
Thematic series Animal reproductive biology Edited by: Prof Fuller Bazer Collection published: 26 March 2015
Thematic series Special Issue for WCAP 2013 Edited by: Prof Defa Li Collection published: 30 August 2013
Thematic series Special Issue for Chinese Swine Industry Symposium Collection published: 8 July 2013
Thematic series Animal Production Collection published: 14 March 2013
Thematic series Reproduction and physiology Collection published: 14 March 2013
Thematic series Animal Nutrition Collection published: 14 March 2013
Thematic series Animal Genetics Collection published: 14 March 2013
Featured series
Pan-genome research in farm animal science
Article collections
Advances in silage research
Gut microbiome and animal health
Poultry genetics and genomics
Interplay between feed, additives, and gut health of monogastric animals
Nutritional modulation of embryonic development and potential in livestock and poultry
View all article collections
Aims and scope
Journal of Animal Science and Biotechnology is an open access, peer-reviewed journal that encompasses a wide range of research areas including animal genetics, reproduction, nutrition, physiology, biochemistry, biotechnology, feedstuffs and animal products. The journal publishes original and novel research articles and reviews mainly involved in pigs, poultry, beef cattle, cows, goats and sheep, but the studies involving aquatic and laboratory animal species that address fundamental questions related to livestock are also welcome.
- Editorial Board
- Manuscript editing services
- Instructions for Editors
- Sign up for article alerts and news from this journal
- Follow us on Twitter
Annual Journal Metrics
Citation Impact 2023 Journal Impact Factor: 6.3 5-year Journal Impact Factor: 6.4 Source Normalized Impact per Paper (SNIP): 1.647 SCImago Journal Rank (SJR): 1.453
Speed 2023 Submission to first editorial decision (median days): 7 Submission to acceptance (median days): 119
Usage 2023 Downloads: 1,188,041 Altmetric mentions: 583
- More about our metrics
ISSN: 2049-1891
- Submission enquiries: Access here and click Contact Us
- General enquiries: [email protected]

An official website of the United States government
The .gov means it’s official. Federal government websites often end in .gov or .mil. Before sharing sensitive information, make sure you’re on a federal government site.
The site is secure. The https:// ensures that you are connecting to the official website and that any information you provide is encrypted and transmitted securely.
- Publications
- Account settings
The PMC website is updating on October 15, 2024. Learn More or Try it out now .
- Advanced Search
- Journal List
- Elsevier - PMC COVID-19 Collection

Animal tissue culture principles and applications
1 Department of Plant Pathology, Institute of Plant Breeding Genetics & Genomics, Center for Applied Genetic Technologies, University of Georgia, Athens, GA, United States
Megha Verma
2 College of Arts and Sciences, St. Louis, MO, United States
Anchal Singh
3 Department of Biochemistry, Institute of Science, Banaras Hindu University, Varanasi, UP, India
Animal cell culture technology in today’s scenario has become indispensable in the field of life sciences, which provides a basis to study regulation, proliferation, and differentiation and to perform genetic manipulation. It requires specific technical skills to carry out successfully. This chapter describes the essential techniques of animal cell culture as well as its applications.
What you can expect to know
This chapter describes the basics of animal cell culture along with the most recent applications. The primary aim is to progressively guide students through fundamental areas and to demonstrate an understanding of basic concepts of cell culture as well as how to perform cell cultures and handle cell lines. This chapter gives insights into types of cell culture, culture media and use of serum, viability assays, and the translational significance of cell culture.
History and methods
Introduction.
Cell culture is the process by which human, animal, or insect cells are grown in a favorable artificial environment. The cells may be derived from multicellular eukaryotes, already established cell lines or established cell strains. In the mid-1900s, animal cell culture became a common laboratory technique, but the concept of maintaining live cell lines separated from their original tissue source was discovered in the 19th century. Animal cell culture is now one of the major tools used in the life sciences in areas of research that have a potential for economic value and commercialization. The development of basic culture media has enabled scientists to work with a wide variety of cells under controlled conditions; this has played an important role in advancing our understanding of cell growth and differentiation, identification of growth factors, and understanding of mechanisms underlying the normal functions of various cell types. New technologies have also been applied to investigate high cell density bioreactor and culture conditions.
Many products of biotechnology (such as viral vaccines) are fundamentally dependent on mass culturing of animal cell lines. Although many simpler proteins are being produced using rDNA in bacterial cultures, more complex proteins that are glycosylated (carbohydrate-modified) currently have to be made in animal cells. At present, cell culture research is aimed at investigating the influence of culture conditions on viability, productivity, and the constancy of post-translational modifications such as glycosylation, which are important for the biological activity of recombinant proteins. Biologicals produced by recombinant DNA (rDNA) technology in animal cell cultures include anticancer agents, enzymes, immunobiologicals [interleukins, lymphokines, monoclonal antibodies (mABs)], and hormones.
Animal cell culture has found use in diverse areas, from basic to advanced research. It has provided a model system for various research efforts:
- 1. The study of basic cell biology, cell cycle mechanisms, specialized cell function, cell–cell and cell–matrix interactions.
- 2. Toxicity testing to study the effects of new drugs.
- 3. Gene therapy for replacing nonfunctional genes with functional gene-carrying cells.
- 4. The characterization of cancer cells, the role of various chemicals, viruses, and radiation in cancer cells.
- 5. Production of vaccines, mABs, and pharmaceutical drugs.
- 6. Production of viruses for use in vaccine production (e.g., chicken pox, polio, rabies, hepatitis B, and measles).
Today, mammalian cell culture is a prerequisite for manufacturing biological therapeutics such as hormones, antibodies, interferons, clotting factors, and vaccines.
Development of animal cell culture
The first mammalian cell cultures date back to the early 20th century. The cultures were originally created to study the development of cell cultures and normal physiological events such as nerve development. Ross Harrison in 1907 showed the first nerve fiber growth in vitro. However, it was in the 1950s that animal cell culture was performed at an industrial scale. It was with major epidemics of polio in the 1940s and 1950s and the accompanying requirement for viral vaccines that the need for cell cultures on a large scale became apparent. The polio vaccine from a de-activated virus became one of the first commercial products developed from cultured animal cells ( Table 14.1 ).
Milestones in cell cultures and microfluidics.
1878 | Claude Bernard | Established that a physiological state of the cell similar to the live cell can be maintained even after the death of the organism. |
1907 | Harrison | Cell entrapment and frog embryo nerve fiber growth in vitro. |
1912 | Alexis Carriel | Initiated tissue culture of chick embryo heart cells using embryo extracts as cultural media passaged for a reported period of 34 years. |
1913 | Steinhardt, Israeli, and Lambert | Grew vaccinia virus in fragments of guinea pig corneal tissue. |
1916 | Rous and Jone | Used trypsin to suspend attached cells in culture. |
1927 | Carrel and Rivera | First viral vaccine against chicken pox. |
1948 | Sanford, Earle, and Likely | Single-cell culture using microscale glass capillaries |
1949 | Enders, Weller, and Robbins | Polio virus grown on human embryonic cells in culture. |
1952 | Gey | Establishment of continuous cell line from a human cervical carcinoma (HeLa cells). |
1955 | Eagle | Established nutrient requirements of cells in culture and defined culture media for growth. |
1956 | Little Field | HAT (hypoxanthin, aminopterin, thymidine) medium introduced for cell selection. |
1961 | Hayflick and Moorhead | Studied human fibroblasts (WI-38) and showed finite lifespan in culture. |
1965 | Ham | First serum-free HAMS’s media. |
1975 | Kohler and Milstein | First hybridoma secreting a monoclonal antibody. |
1977 | Genetech | First recombinant human protein: somatistatin. |
1979 | Terry, Jerman, and Angell | Gas chromatography system using silicon-etched microchannels |
1985 | Collen | Recombinant tissue plasminogen activator (tPA) in mammalian cells. Human growth hormone produced from recombinant bacteria was accepted for therapeutic use. |
1986 | FDA approval | First monoclonal antibody was approved by the FDA for use in humans (Orthoclone OKT3). |
1986 | Genetech | First recombinant protein commercialized (interferon alpha-2a). |
1989 | Amgen | Erythropoietin (EPO) recombinant protein produced in CHO cells available commercially. |
1992 | Manz, Harrison, Verpoorte, Fettinger, Paulus, Ludi, and Widmer | Micro-machined glass chip electrophoresis for separating molecules |
1992 | FDA approval | First genetically engineered recombinant blood-clotting factor used in the treatment of hemophilia A. |
1996 | Wilmut | Production of transgenic sheep (Dolly) through nuclear transfer technique. |
1997 | Hadd, Raymond, Halliwell, Jacobson, and Ramsey | Microchip device for enzyme assay |
1998 | Duffy, McDonald, Schueller, and Whitesides | Creation of microfluidic systems using PDMS (polydimethylsiloxane) |
2000 | Panaro, Yuen, Sakazume, Fortina, Kricka, Wilding | Lab-on-chip system (bioanalyzer) |
2002 | Cloneaid | Claimed to produce cloned human baby named EVE. |
2003 | Zheng | Protein crystallization chip |
2004 | FDA approval | First antiangiogenic monoclonal antibody that inhibits the growth of blood vessels or angiogenesis (for cancer therapy). |
2005 | Sanford, Earle, and Likely | Single-cell culture in open microfluidic systems |
2005 | Birch | Reported antibody titers at an industrial scale of 5 g/L and more. |
2009 | Nathalie Cartier-Lacave | Combined gene therapy with blood stem cell therapy, which may be a useful tool for treating fatal brain disease. |
2011 | Melanie Welham, David Tosh | 1 M molecule treatment causes stem cells to turn into precursors of liver cells. |
2012 | Willison and Klug | Single-cell and single-molecule proteomics utilizing nanospace technology |
2012 | Maria Blasco | First gene therapy successful against aging-associated decline in mice. |
Basic concept of cell culture
Tissue culture is in vitro maintenance and propagation of isolated cells tissues or organs in an appropriate artificial environment. Many animal cells can be induced to grow outside of their organ or tissue of origin under defined conditions when supplemented with a medium containing nutrients and growth factors. For in vitro growth of cells, the culture conditions may not mimic in vivo conditions with respect to temperature, pH, CO 2 , O 2 , osmolality, and nutrition. In addition, the cultured cells require sterile conditions along with a steady supply of nutrients for growth and sophisticated incubation conditions. An important factor influencing the growth of cells in culture medium is the medium itself. At present, animal cells are cultured in natural media or artificial media depending on the needs of the experiment. The culture medium is the most important and essential step in animal tissue culture. This depends on the type of cells that need to be cultured for the purpose of cell growth differentiation or production of designed pharmaceutical products. In addition, serum-containing and serum-free media are now available that offer a varying degree of advantage to the cell culture. Sterile conditions are important in the development of cell lines.
Cells from a wide range of different tissues and organisms are now grown in the lab. Earlier, the major purpose of cell culture was to study the growth, the requirements for growth, the cell cycle, and the cell itself. At present, homogenous cultures obtained from primary cell cultures are useful tools to study the origin and biology of the cells. Organotypic and histotypic cultures that mimic the respective organs/tissues have been useful for the production of artificial tissues.
How are cell cultures obtained?
There are three methods commonly used to initiate a culture from animals.
Organ culture
Whole organs from embryos or partial adult organs are used to initiate organ culture in vitro. These cells in the organ culture maintain their differentiated character, their functional activity, and also retain their in vivo architecture. They do not grow rapidly, and cell proliferation is limited to the periphery of the explant. As these cultures cannot be propagated for long periods, a fresh explanation is required for every experiment that leads to interexperimental variation in terms of reproducibility and homogeneity. Organ culture is useful for studying functional properties of cells (production of hormones) and for examining the effects of external agents (such as drugs and other micro or macro molecules) and products on other organs that are anatomically placed apart in vivo.
Primary explant culture
Fragments exercised from animal tissue may be maintained in a number of different ways. The tissue adheres to the surface aided by an extracellular matrix (ECM) constituent, such as collagen or a plasma clot, and it can even happen spontaneously. This gives rise to cells migrating from the periphery of the explant. This culture is known as a primary explant, and migrating cells are known as outgrowth. This has been used to analyze the growth characteristics of cancer cells in comparison to their normal counterparts, especially with reference to altered growth patterns and cell morphology.
Cell culture
This is the most commonly used method of tissue culture and is generated by collecting the cells growing out of explants or dispersed cell suspensions (floating free in culture medium). Cells obtained either by enzymatic treatment or by mechanical means are cultured as adherent monolayers on solid substrate.
Cell culture is of three types: (1) precursor cell culture, which is undifferentiated cells committed to differentiate; (2) differentiated cell culture, which is completely differentiated cells that have lost the capacity to further differentiate; and (3) stem cell culture, which is undifferentiated cells that go on to develop into any type of cell.
Cells with a defined cell type and characteristics are selected from a culture by cloning or by other methods; this cell line becomes a cell strain.
Monolayer cultures
The monolayer culture is an anchorage-dependent culture of usually one cell in thickness with a continuous layer of cells at the bottom of the culture vessel.
Suspension cultures
Some of the cells are nonadhesive and can be mechanically kept in suspension, unlike most cells that grow as monolayers (e.g., cells of leukemia). This offers numerous advantages in the propagation of cells.
Cell passage and use of trypsin
Passaging is the process of subculturing cells in order to produce a large number of cells from pre-existing ones. Subculturing produces a more homogeneous cell line and avoids the senescence associated with prolonged high cell density. Splitting cells involves transferring a small number of cells into each new vessel. After subculturing, cells may be propagated, characterized, and stored. Adherent cell cultures need to be detached from the surface of the tissue culture flasks or dishes using proteins. Proteins secreted by the cells form a tight bridge between the cell and the surface. A mixture of trypsin-EDTA is used to break proteins at specific places. Trypsin is either protein-degrading or proteolytic; it hydrolyzes pepsin-digested peptides by hydrolysis of peptide bonds. EDTA sequesters certain metal ions that can inhibit trypsin activity, and thus enhances the efficacy of trypsin. The trypsinization process and procedure to remove adherent cells is given in Flowchart 14.1 .

Trypsinization of adherent cells.
Quantitation
Quantitation is carried out to characterize cell growth and to establish reproducible culture conditions.
Hemocytometer
Cell counts are important for monitoring growth rates as well as for setting up new cultures with known cell numbers. The most widely used type of counting chamber is called a hemocytometer. It is used to estimate cell number. The concentration of cells in suspension is determined by placing the cells in an optically clear chamber under a microscope. The cell number within a defined area of known depth is counted, and the cell concentration is determined from the count.
Electronic counting
For high-throughput work, electronic cell counters are used to determine the concentration of each sample.
Other quantitation
In some cases, the DNA content or the protein concentration needs to be determined instead of the number of cells.
Reconstruction of three-dimensional structures
Cells propagated as a cell suspension or monolayer offer many advantages but lack the potential for cell-to-cell interaction and cell–matrix interaction seen in organ cultures. For this reason, many culture methods that start with a dispersed population of cells encourage the arrangement of these cells into organ-like structures. These types of cultures can be divided into two basic types.
Histotypic culture
Cell–cell interactions similar to tissue-like densities can be attained by the use of an appropriate ECM and soluble factors and by growing cell cultures to high cell densities. This can be achieved by (a) growing cells in a relatively large reservoir with adequate medium fitted with a filter where the cells are crowded; (b) growing the cells at high concentrations on agar or agarose or as stirred aggregates (spheroids); and (c) growing cells on the outer surface of hollow fibers where the cells are seeded on the outer surface and medium is pumped through the fibers from a reservoir.
Organotypic culture
To simulate heterotypic cell interactions in addition to homotypic cell interactions, cells of differentiated lineages are re-combined. Co-culturing of epithelial and fibroblast cell clones from the mammary gland allows the cells to differentiate functionality under the correct hormonal environment, thus producing milk proteins.
Types of cell culture
Primary cell culture.
These cells are obtained directly from tissues and organs by mechanical or chemical disintegration or by enzymatic digestion. These cells are induced to grow in suitable glass or plastic containers with complex media. These cultures usually have a low growth rate and are heterogeneous; however, they are still preferred over cell lines as these are more representative of the cell types in the tissues from which they are derived. The morphological structure of cells in culture is of various types: (1) epithelium type, which are polygonal in shape and appear flattened as they are attached to a substrate and form a continuous thin layer (i.e., monolayer on solid surfaces); (2) epitheloid type, which have a round outline and do not form sheets like epithelial cells and do not attach to the substrate; (3) fibroblast type, which are angular in shape and elongated and form an open network of cells rather than tightly packed cells, are bipolar or multipolar, and attach to the substrate; and (4) connective tissue type, which are derived from fibrous tissue, cartilage, and bone, and are characterized by a large amount of fibrous and amorphous extracellular materials.
Advantages and disadvantages of primary cell culture
These cultures represent the best experimental models for in vivo studies. They share the same karyotype as the parent and express characteristics that are not seen in cultured cells. However, they are difficult to obtain and have limited lifespans. Potential contamination by viruses and bacteria is also a major disadvantage.
Depending on the kind of cells in culture, the primary cell culture can also be divided into two types.
Anchorage-dependent/adherent cells
These cells require a stable nontoxic and biologically inert surface for attachment and growth and are difficult to grow as cell suspensions. Mouse fibroblast STO cells are anchorage cells.
Anchorage-independent/suspension cells
These cells do not require a solid surface for attachment or growth. Cells can be grown continuously in liquid media. The source of cells is the governing factor for suspension cells. Blood cells are vascular in nature and are suspended in plasma and these cells can be very easily established in suspension cultures.
Secondary cell culture
When primary cell cultures are passaged or subcultured and grown for a long period of time in fresh medium, they form secondary cultures and are long-lasting (unlike cells of primary cell cultures) due to the availability of fresh nutrients at regular intervals. The passaging or subculturing is carried out by enzymatic digestion of adherent cells. This is followed by washing and re-suspending of the required amount of cells in appropriate volumes of growth media. Secondary cell cultures are preferred as these are easy to grow and are readily available; they have been useful in virological, immunological, and toxicological research.
Advantages and disadvantages of secondary cell culture
This type of culture is useful for obtaining a large population of similar cells and can be transformed to grow indefinitely. These cell cultures maintain their cellular characteristics. The major disadvantage of this system is that the cells have a tendency to differentiate over a period of time in culture and generate aberrant cells.
The primary culture, when subcultured, becomes a cell line or cell strain that can be finite or continuous, depending on its lifespan in culture. They are grouped into two types on the basis of the lifespan of the culture.
Finite cell lines
Cell lines with a limited number of cell generations and growth are called finite cell lines. The cells are slow growing (24–96 hours). These cells are characterized by anchorage dependence and density limitation.
Indefinite cell lines
Cell lines obtained from in vitro transformed cell lines or cancerous cells are indefinite cell lines and can be grown in monolayer or suspension form. These cells divide rapidly with a generation time of 12–14 hours and have a potential to be subcultured indefinitely. The cell lines may exhibit aneuploidy ( Bhat, 2011 ) or heteroploidy due to an altered chromosome number. Immortalized cell lines are transformed cells with altered growth properties. HeLa cells are an example of an immortal cell line. These are human epithelial cells obtained from fatal cervical carcinoma transformed by human papilloma virus 18 (HPV18). Indefinite cell lines are easy to manipulate and maintain. However, these cell lines have a tendency to change over a period of time.
Commonly used cell lines
Nowadays, for the production of biologically active substances on an industrial scale, a mammalian cell culture is a prerequisite. With advancements in animal cell culture technology, a number of cell lines have evolved and are used for vaccine production, therapeutic proteins, pharmaceutical agents, and anticancerous agents. For the production of cell lines, human, animal, or insect cells may be used. Cell lines that are able to grow in suspension are preferred as they have a faster growth rate. Chinese hamster ovary (CHO) is the most commonly used mammalian cell line.
When selecting a cell line, a number of general parameters must be considered, such as growth characteristics, population doubling time, saturation density, plating efficiency, growth fraction, and the ability to grow in suspension. Table 14.2 shows some of the commonly used cell lines.
Commonly used cell lines and their origins.
Cell line | Origin | Organism |
---|---|---|
H1, H9 | Embryonic stem cells | Human |
HEK-293 | Embryonic kidney transformed with adenovirus | Human |
HeLa | Epithelial cell | Human |
HL 60 | Human promyelocytic leukemia cells | Human |
MCF-7 | Breast cancer | Human |
A549 | Lung cancer | Human |
A1 to A5-E | Amnion | Human |
ND-E | Esophagus | Human |
CHO | Ovary | Chinese hamster |
Vero | Kidney epithelial cell | African green monkey |
Cos-7 | Kidney cells | African green monkey |
3T3 | Fibroblast | Mouse |
BHK21 | Fibroblast | Syrian hamster |
MDCK | Epithelial cell | Dog |
E14.1 | Embryonic stem cells (mouse) | Mouse |
COS | Kidney | Monkey |
DT40 | Lymphoma cell | Chick |
S2 | Macrophage-like cells | Drosophila |
GH3 | Pituitary tumor | Rat |
L6 | Myoblast | Rat |
Sf9 and Sf21 | Ovaries | Fall Army worm( ) |
ZF4 and AB9 cells | Embryonic fibroblast cells | Zebrafish |
Advantages of continuous cell lines
- 1. Continuous cell lines show faster cell growth and achieve higher cell densities in culture.
- 2. Serum-free and protein-free media for widely used cell lines may be available in the market.
- 3. The cell lines have a potential to be cultured in suspension in large-scale bioreactors.
The major disadvantages of these cultures are chromosomal instability, phenotypic variation in relation to the donor tissue, and a change in specific and characteristic tissue markers ( Freshney, 1994 ).
Growth cycle
The cells in the culture show a characteristic growth pattern, lag phase, exponential or log phase, followed by a plateau phase. The population doubling time of the cells can be calculated during the log phase and plateau phase. This is critical and can be used to quantify the response of the cells to different culture conditions for changes in nutrient concentration and effects of hormonal or toxic components. The population doubling time describes the cell division rates within the culture and is influenced by nongrowing and dying cells.
Phases of the growth cycle
The population doubling time, lag time, and saturation density of a particular cell line can be established and characterized for a particular cell type. A growth curve consists of a normal culture and can be divided into a lag phase, log phase, and plateau phase.
This is the initial growth phase of the subculture and re-seeding during which the cell population takes time to recover. The cell number remains relatively constant prior to rapid growth. During this phase, the cell replaces elements of the glycocalyx lost during trypsinization, attaches to the substrate, and spreads out. During the spreading process, the cytoskeleton reappears; its reappearance is probably an integral part of the process.
This is a period of exponential increase in cell number and growth of the cell population due to continuous division. The length of the log phase depends on the initial seeding density, the growth rate of the cells, and the density at which cell proliferation is inhibited by density. This phase represents the most reproducible form of the culture as the growth fraction and viability is high (usually 90%–100%), and the population is at its most uniform. However, the cell culture may not be synchronized, and the cells can be randomly distributed in the cell cycle.
Plateau phase
The culture becomes confluent at the end of the log phase as growth rates during this phase are reduced, and cell proliferation can cease in some cases due to exhaustion. The cells are in contact with surrounding cells, and the growth surface is occupied. At this stage, the culture enters the stationary phase and the growth fraction falls to between 0% and 10%. Also, the constitution and charge of the cell surface may be changed, and there may be a relative increase in the synthesis of specialized versus structural proteins.
Monitoring cell growth
The animal cell culture can be grown for a wide variety of cell-based assays to investigate morphology, protein expression, cell growth, differentiation, apoptosis, and toxicity in different environments. Product yields can be increased if monitoring of cell growth is managed properly. A number of factors affect the maximum growth of cells in a batch reactor. Regular observation of cells in culture helps monitor cell health and the stage of growth; small changes in pH, temperature, humidity, O 2 , CO 2 , dissolved nutrients, etc., could have an impact on cell growth. Monitoring the rate of growth continuously also provides a record that the cells have reached their maximum density within a given time frame.
Characteristics of cell cultures
Animal cell cultures show specific characteristics and differ from microbial cultures. The important characteristics of the animal cell are slow growth rate, requirement of solid substrata for anchorage-dependent cells, lack of a cell wall (which leads to fragility), and sensitivity to physiochemical conditions such as pH, CO 2 levels, etc. Some of the fundamental bioprocess variables are as follows:
Temperature
Temperature is one of the most fundamental variables as it directly interferes with the growth and production processes. On a small scale, thermostatically controlled incubators can be used to control temperature. However, cell cultures grown on a large scale in bioreactors require more sensitive control of temperature. Different bioreactors use different methods to maintain the temperature of the cell culture. Temperature in a bioreactor is maintained by a heat blanket and water jacket with a temperature sensor.
pH of the culture medium can be controlled by adding alkali (NaOH, KOH) or acid (HCl) solution. Addition of CO 2 gas to the bioreactor, buffering with sodium bicarbonate, or use of naturally buffering solutes help maintain the pH of the culture. A silver chloride electrochemical-type pH electrode is the most commonly used electrode in the bioreactor.
Dissolved oxygen is the most fundamental variable that needs to be continuously supplied to the cell culture medium. It is consumed with a carbon source in aerobic cultures ( Moore et al., 1995 ). Diffusion through a liquid surface or membranes is one of the methods for providing dissolved oxygen to the medium.
Cell viability
The number of viable cells in the culture provides an accurate indication of the health of the cell culture ( Stacey and Davis, 2007 ). Trypan blue and erythrosin B determine cell viability through the loss of cellular membrane integrity. Both these dyes are unable to penetrate the cell membrane when the membrane is intact, but are taken up and retained by dead cells (which lack an intact membrane). Erythrosin B stain is preferred over Trypan blue as it generates more accurate results with fewer false negatives and false positives.
Cytotoxicity
The toxic chemicals in the culture medium affect the basic functions of cells. The cytotoxicity effect can lead to the death of the cells or alterations in their metabolism. Methods to access viable cell number and cell proliferation rapidly and accurately is the important requirement in many experimental situations that involve in vitro and in vivo studies. The cell number determination can be useful for determining the growth factor activity, concentration of toxic compound, drug screening, duration of exposure, change in colony size, carcinogenic effects of chemical compounds, and effects of solvents (such as ethanol, propylene, etc.).
The assays to measure viable cells (viability assays) are as follows:
- 1. [3-(4,5-dimethylthiazol-2-yl)-2,5-diphenyltetrazolium bromide] (MTT)/MTS/resazurin assay.
- 2. Protease marker assay.
- 3. ATP assay.
The MTT assay allows simple, accurate, and reliable counting of metabolically active cells based on the conversion of pale yellow tetrazolium MTT. Nicotinamide adenine dinucleotide in metabolically active viable cells reduces tetrazolium compounds into brightly colored formazan products or reduces resazurin into fluorescent resorufin ( Fig. 14.1 ). MTT and resazurin assays are widely used, as they are inexpensive and can be used with all cell types. The protease marker assay utilizes the cell-permeant protease substrate glycylphenylalanyl-aminofluorocoumarin (GF-AFC). The substrate, which lacks an aminoterminal blocking moiety, is processed by aminopeptidases within the cytoplasm to release AFC. The amount of AFC released is proportional to the viable cell number. This assay has better sensitivity than resuzurin and the cells remain viable; thus, multiplexing is possible. The ATP assay is the most sensitive cell viability assay. It is measured using the beetle luciferase reaction to generate light. The MTT assay and procedure is given in Flowchart 14.2 .

Schematic summary of biochemical events in different viability assays.

Assays to detect dead cells are as follows:
- 1. Lactate dehydrogenase (LDH) release.
- 2. Protease release.
- 3. DNA staining.
The viable cells in culture have intact outer membranes. Loss of membrane integrity defines a “dead” cell. The dead cells can be detected by measuring the activity of marker enzymes that leak out of dead cells into the culture medium or by staining the cytoplasmic or nuclear content by vital dyes that can only enter dead cells. LDH is an enzyme that is present in all cell types. It catalyzes the oxidation of lactate to pyruvate in the presence of co-enzyme NAD + . In the damaged cells, LDH is rapidly released. The amount of released LDH is used to assess cell death ( Fig. 14.2 ). This assay is widely used but has limited sensitivity as half-life of LDH at 37 °C is 9 hours.

Principle of the LDH release assay.
The protease release assay is based on the intracellular release of proteases from the dead/compromised cell into the culture medium. The released proteases cleave the substrate to liberate aminoluciferin, which serves as a substrate for luciferase ( Fig. 14.3 ) and leads to the production of a “glowtype” signal ( Cho et al., 2008 ).

Principle of the luminescent protease release assay.
Hayflick’s phenomenon
Hayflick limit or Hayflick’s phenomena is defined as the number of times a normal cell population divides before entering the senescence phase. Macfarlane Burnet coined the term “c limit” in 1974. Hayflick and Moorhead (1961) demonstrated that a population of normal human fetal cells divide in culture between 40 and 60 times before stopping. There appears to be a correlation between the maximum number of passages and aging. This phenomenon is related to telomere length. Repeated mitosis leads to shortening of the telomeres on the DNA of the cell. Telomere shortening in humans eventually makes cell division impossible, and correlates with aging. This explains the decrease in passaging of cells harvested from older individuals.
Culture media
One of the most important factors in animal cell culture is the medium composition. In vitro growth and maintenance of animal cells require appropriate nutritional, hormonal, and stromal factors that resemble their milieu in vivo as closely as possible. Important environmental factors are the medium in which the cells are surrounded, the substratum upon which the cells grow, temperature, oxygen and carbon dioxide concentration, pH, and osmolality. In addition, the cell requires chemical substances that cannot be synthesized by the cells themselves. Any successful medium is composed of isotonic, low-molecular-weight compounds known as basal medium and provides inorganic salts, an energy source, amino acids, and various supplements.
Basic components in culture media
The 10 basic components that make up most of the animal cell culture media are as follows: inorganic salts (Ca 2+ , Mg 2+ , Na + , K + ), nitrogen source (amino acids), energy sources (glucose, fructose), vitamins, fat and fat soluble component (fatty acids, cholesterols), nucleic acid precursors, growth factors and hormones, antibiotics, pH and buffering systems, and oxygen and carbon dioxide concentrations.
Complete formulation of media that supports growth and maintenance of a mammalian cell culture is very complex. For this reason, the first culture medium used for cell culture was based on biological fluids such as plasma, lymph serum, and embryonic extracts. The nutritional requirements of cells can vary at different stages of the culture cycle. Different cell types have highly specific requirements, and the most suitable medium for each cell type must be determined experimentally. Media may be classified into two categories: (1) natural media and (2) artificial media.
Natural media
Natural media consist of naturally occurring biological fluids sufficient for the growth and proliferation of animals cells and tissues. This media useful for promoting cell growth are of the following three types:
- 1. Coagulant or clots: Plasma separated from heparinized blood from chickens or other animals is commercially available in the form of liquid plasma.
- 2. Biological fluids: This includes body fluids such as plasma, serum lymph, amniotic fluid, pleural fluid, insect hemolymph, and fetal calf serum. These fluids are used as cell culture media after testing for toxicity and sterility.
- 3. Tissue extract: Extracts of liver, spleen, bone marrow, and leucocytes are used as cell culture media. Chicken embryo extract is the most common tissue extract used in some culture media.
Artificial media
The media contains partly or fully defined components that are prepared artificially by adding several nutrients (organic and inorganic). It contains a balanced salt solution with specific pH and osmotic pressure designed for immediate survival of cells. Artificial media supplemented with serum or with suitable formulations of organic compounds supports prolonged survival of the cell culture.
The artificial media may be grouped into the following four classes: serum-containing media, serum-free media, chemically defined media, and protein-free media.
The clear yellowish fluid obtained after fibrin and cells are removed from blood is known as serum. It is an undefined media supplement of extremely complex mixture of small and large molecules and contains amino acids, growth factors, vitamins, proteins, hormones, lipids, and minerals, among other components ( Table 14.3 ).
Serum components, their composition, and role in animal cell culture.
Component | Probable function |
---|---|
Albumin | Major binding and buffering agent, antioxidant, transporter of insoluble molecules |
Transferrin | Iron chelator and transporter |
Alpha, beta, and gamma globulin fractions | Bind to iron and iron carrier and prevent infection |
Regulatory proteins | Regulate gene expression |
Epidermal growth factor (EGF) | Proliferation and differentiation |
Fibroblast growth factor (FGF) | Proliferation and differentiation |
Insulin | Glucose and protein metabolism |
Transferrin | Incorporation of iron by cells |
Advantages of serum in cell culture medium
- 1. It has basic nutrients present either in soluble or in protein-bound form.
- 2. It provides several hormones such as insulin and transferrin. Insulin is essential for the growth of nearly all cells in culture and transferrin acts as an iron binder.
- 3. It contains numerous growth factors such as platelet-derived growth factor (PDGF), transforming growth factor beta (TGF-B), epidermal growth factor (EGF), and chondronectin. These factors stimulate cell growth and support specialized functions of cells.
- 4. It supplies protein, which helps in the attachment of cells to the culture surface (e.g., fibronectin).
- 5. It provides binding proteins such as albumin and transferrin, which helps transport molecules in cells.
- 6. It provides minerals such as Ca 2+ , Mg 2+ , Fe 2+ , K + Na + , Zn 2+ , etc., which promote cell attachment.
- 7. It increases the viscosity of the medium, which provides protection against mechanical damage during agitation and aeration of suspension cultures.
- 8. It provides appropriate osmotic pressure.
Disadvantages of serum-containing medium
- 1. Expensive: Fetal calf serum is expensive and difficult to obtain in large quantities.
- 2. Variation: Batch-to-batch variation occurs in serum, and there is no uniformity in composition of serum. This can affect growth and yields and can give inconsistent results.
- 3. Contamination: Serum medium carries a high risk of contamination with virus, fungi, and mycoplasma.
- 4. Cytotoxic and inhibiting factors: The serum itself may be cytotoxic and may contain inhibiting factors, which in turn may inhibit cultured cell growth and proliferation. The enzyme polyamine oxidase in serum reacts with polyamines such as spermine and spermidine to form cytotoxic polyamino-aldehyde.
- 5. Downstream processing: The presence of serum in culture media may interfere with isolation and purification of culture products. Additional steps may be required to isolate cell culture products.
Serum-free media
The use of serum in culture media presents a safety hazard and source of unwanted contamination for the production of biopharmaceuticals. As a number of cell lines can be grown in serum-free media supplemented with certain components of bovine fetal serum, the development of this type of medium with a defined composition has intensified in the last few decades. Eagle (1959) developed a “minimal essential medium” composed of balanced salts, glucose, amino acids, and vitamins. In the last 50 years, considerable work has been carried out to develop more efficient culture media to meet the specific requirements of specific cell lines.
Advantages of serum-free culture media
- 1. Serum-free media are simplified, and the composition is better defined.
- 2. They can be designed specifically for a cell type. It is possible to create different media and to switch from growth-enhancing media to differentiation-inducing media by altering the combination and types of growth factors and inducers.
- 3. They decrease variability from batch to batch and improve reproduction between cultures.
- 4. Downstream processing of products from cell cultures in serum-free media is easier.
- 5. They reduce the risk of microbial contamination (mycoplasma, viruses, and prions).
- 6. Serum-free media are easily available and ready to use. They are also cost-effective when compared with serum-containing media.
Disadvantages of serum-free media
- 1. Growth rate and saturation density attained are lower than those compared to serum-containing media.
- 2. Serum-free media prove to be more expensive as supplementing with hormone and growth factors increases the cost enormously.
- 3. Different media are required for different cell types as each species has its own characteristic requirements.
- 4. Critical control of pH and temperature and ultra-purity of reagent and water are required as compared to serum-containing media.
Chemically defined media
These media contain pure inorganic and organic constituents along with protein additions like EGFs, insulin, vitamins, amino acids, fatty acids, and cholesterol.
Protein-free media
These media contain nonprotein constituents necessary for the cell culture. The formulations of DME, MEM, RPMI-1640, ProCHO TM, and CDM-HD are examples of protein-free media. They promote superior cell growth and facilitate downstream purification of expressed products.
Characterization of cell lines
The characterization of cell lines is important to ensure the quality of cell-derived biopharmaceutical products. It helps in determining the cell source with regard to its identity and the presence of other cell lines, molecular contaminants, and endogenous agents. The characterization of mammalian cell lines is species-specific and can vary depending on the history of the cell line and type of media components used for culturing.
Mammalian cell line characterization can be done in four ways:
- 1. Identity testing.
- 2. Purity testing.
- 3. Stability testing.
- 4. Virological safety testing.
Identity testing
Identity testing can be carried out by isoenzyme analysis. The banding pattern of the intracellular enzyme (which is species-specific) can be determined by using agarose gels. DNA fingerprinting and karyotyping, and DNA and RNA sequencing are alternative methods to identity testing.
Karyotyping
Karyotyping is important as it determines any gross chromosomal changes in the cell line. The growth conditions and subculturing of a cell line may lead to alteration in the karyotype; for example, HeLa cells were the first human epithelial cancer cell line established in long-term culture, and they have a hyper-triploid chromosome number (3n1).
Purity testing
Bacterial and fungal contamination of cell lines occurs due to impure techniques and source material. The occurrence of contaminants can be tested by a direct inoculation method on two different media. Mycoplasma infection is the contamination of cell cultures/cell lines with mycoplasmas, and it represents a serious problem. Detection by microscopy is not adequate and requires additional testing by fluorescent staining PCR, ELISA assay, autoradiography, immune-staining, or microbiological assay.
Stability testing
Characterization and testing of cell substrate (cell line derived from human or animal source) is one of the most important components in the control of biological products. It helps to confirm the identity, purity, and suitability of the cell substrate for manufacturing use. The substrate stability should be examined at a minimum of two time points during cultivation for production. In addition, genetic stability can be tested by genomic or transcript sequencing, restriction map analysis, and copy number determination (FDA guidelines, 2012).
Viral testing assays
Virus testing of cell substrate should be designed to detect a spectrum of viruses. Appropriate screening tests should be carried out based on the cultivation history of cell lines. The development of characteristic cytopathogenic effect (CPE) provides an early indication of viral contamination. Some of the viruses of special concern in cell production work are human immunodeficiency virus, human papilloma virus, hepatitis virus, human herpes virus, hantavirus, simian virus, sendai virus, and bovine viral diarrhea virus. For detection of viruses causing immunodeficiency diseases and hepatitis, detection of sequences by PCR testing is adequate. Cells exposed to be serum or bovine serum albumin require a bovine virus test. Some of the viral testing assays are XC plaque assays, S+L-focus assay, reverse transcription assay. XC plaque assay is utilized to detect infectious ecotropic murine retroviruses. S+L-focus assay is used to test cells for the presence of infectious xenotropic and amphotropic murine retroviruses that are capable of interacting with both murine and nonmurine cells. Real-time (RT) assays such as real-time fluorescent product-enhanced reverse transcriptase (FPERT) assay and quantitative real-time for fluorescent product-enhanced reverse transcript (QPERT) assay detect the conversion of an RNA template to cDNA due to the presence of the RT template when retrovirus infection is present in the cell line.
Advantages of animal cell culture
- 1. Physiochemical and physiological condition: Role and effect of pH, temperature, O 2 /CO 2 concentration, and osmotic pressure of the culture media can be altered to study their effects on the cell culture ( Freshney, 2010 ).
- 2. Metabolism of cell: To study cell metabolism and investigate the physiology and biochemistry of cells.
- 3. Cytotoxic assay: Effect of various compounds or drugs on specific cell types such as liver cells can be studied.
- 4. Homogenous cultures: These cultures help study the biology and origin of the cells.
- 5. Valuable biological data from large-scale cell cultures: Specific proteins can be synthesized in large quantities from genetically modified cells in large-scale cultures.
- 6. Consistency of results: Reproducibility of the results that can be obtained by the use of a single type/clonal population.
- 7. Identification of cell type: Specific cell types can be detected by the presence of markers such as molecules or by karyotyping.
- 8. Ethics: Ethical, moral, and legal questions for utilizing animals in experiments can be avoided.

Disadvantages of animal cell culture
- 1. Expenditure and expertise: This is a specialized technique that requires aseptic conditions, trained personnel, and costly equipment.
- 2. Dedifferentiation: Cell characteristics can change after a period of continuous growth of cells in cultures, leading to differentiated properties compared to the original strain.
- 3. Low amount of product: The miniscule amount of mAB and recombinant protein produced followed by downstream processing for extracting pure products increases expenses tremendously.
- 4. Contamination: Mycoplasma and viral infection are difficult to detect and are highly contagious.
- 5. Instability: Aneuploidy chromosomal constitution in continuous cell lines leads to instability.
In addition, this system cannot replace the complex live animal for testing the response of chemicals or the impact of vaccines or toxins.
Ethical issues
Despite considerable progress in the development of cell culture techniques, the potential biohazards of working with animal and human tissues presents a number of ethical problems, including issues of procurement, handling, and ultimate use of material. In most countries, biomedical research is strictly regulated. Legislation varies considerably in different countries. Research ethics committees, animal ethics committees for animal-based research, and institutional research boards for human subjects have a major role in research governance.
Some guidelines for the use of experimental or donor animals include assurances of proper conditions for housing animals and minimal pain or discomfort to any animal that is put to death or operated upon. These guidelines apply to higher vertebrates and not to lower vertebrates such as fish or other invertebrates.
Use of fetal bovine serum in animal culture of media
Fetal bovine serum (FBS)-supplemented media are commonly used in animal cell cultures. In recent years, FBS production methods have come under scrutiny because of animal welfare concerns. FBS is harvested from bovine fetuses taken from pregnant cows during slaughter. The common method of harvesting the fetus is by cardiac puncture without any anesthesia. This practice of harvesting FBS is inhumane as it exposes the fetus to pain and/or discomfort. In addition to moral concerns, numerous scientific and technical problems exist with regard to the use of FBS in cell culture. Efforts are now being made to reduce the use of FBS and replace it with synthetic alternatives.
In the case of human tissues, some considerations that need to be addressed are as follows ( Freshney, 2011 ):
- 1. Consent: Patient’s and/or relative’s approval of tissue use.
- 2. Project summary: Explanation of the project, including the purpose, outcome, and medical benefits of the research.
- 3. Permission requests: Paperwork regarding possible use of the tissues.
- 4. Ownership: Establishment of ownership with regard to cell lines and their derivatives.
- 5. Patent issues: Commercial use of the tissues.
Translational significance
In biomedical research, the use of animal and human cell cultures has become beneficial for diverse applications. It provides indispensable tools for producing a number of products, including biopharmaceuticals, mABs, and products for gene therapy. In addition, animal cell cultures provide adequate test systems for studying biochemical pathways, intra- and intercellular responses, pathological mechanisms, and virus production. Some of the applications of animal cell culture are discussed below.
Antiviral vaccines
Animal cell culture technology has played an important role in the development of viral vaccine production. The establishment of cell culture technology in the 1950s and the consequent replacement of live animals for the development of antigens have led to considerable progress in bioprocess technology. With the advent of DNA technology, molecular manipulation of viruses has led to the development of a recombinant vaccine against hepatitis B virus (HBV) and several others potential vaccines that are in the final phase of clinical trials. Table 14.4 lists recombinant hepatitis B vaccines in eukaryotic cells.
Recombinant hepatitis B vaccines in eukaryotic cells.
Trade name | Manufacture | Recombinant protein | Expression host |
---|---|---|---|
Sci B-Vac | SciGen | HBsAgS, M, and L protein | Mammalian cells (CHO) |
GenHevac B | Pasteur-Merieux Aventis | HBsAg S and M protein | Mammalian cells (CHO) |
Enivac HB | Panacea Biotec Ltd | HBsAg S protein | Yeast ( ) |
Revac-B | Bharat Biotech International Ltd | HBsAg S protein | Yeast ( ) |
Viral particles production by cell culture
Viral particle production by cell culture differs from the production of molecules such as proteins, enzymes, and toxins by bacteria or animal cells. The product formation may not be related to the development or growth of a cell and may occur through secondary metabolic pathways, unlike virus production, which does not result from secondary metabolic pathway. Virus production occurs after the viral infection directs cell machinery to perform viral particle production.
Two stages are involved in viral production:
- 1. Cell culture system: This requires the development of an efficient system for conversion of the culture medium substrate in the cell mass.
Cell lines used for viral vaccine production.
Cell lines | Origin |
---|---|
BHK-21 | Kidney (hamster) |
Vero | Kidney (African green monkey) |
MDCK | Kidney (cocker spaniel) |
CHO | Chinese hamster |
Hela | Epithelial cells (human) |
Production of virus-like particles
Most of the existing classical vaccines for viral disease are either altered or chemically inactive live viruses. However, incomplete inactivation of a virus or reversion of an attenuated strain can risk infection in vaccinated individuals. Viruses with segmented genomes with a high degree of genetic exchange can undergo re-assortment or recombination of genetic material with viruses of different serotypes in the vaccinated host, which can result in the production of new variants of the virus. Moreover, some live virus vaccines are teratogenic; for example, Smithburn neurotropic strain (SNS) ( Smithburn, 1949 ) and MP12-attenuated ( Caplen et al., 1985 ) vaccine strains of the Rift Valley fever virus. A new type of vaccine that does not present the typical side effects of an attenuated or inactivated viral vaccine has been made possible with the development of rDNA technology. Virus-like particles (VLPs) are highly effective as they mimic the overall structure of the virus; however, these particles lack the infectious genetic material. Capsid proteins can aggregate to form core-like particles in the absence of nucleic acids. These spontaneously assembled particles are structurally similar to authenticate viruses and are able to stimulate B-cell-mediated immune responses. In addition, VLPs stimulate a CD4-proliferative response and cytotoxic T-lymphocyte response ( Jeoung et al., 2011 ).
VLPs resemble and mimic virus structure and are able to elicit a strong immune response without causing harm. The major advantage of VLPs is their simplicity and nonpathogenic nature. They are replication-deficient as they lack any viral genetic information, thus eliminating the need for inactivation of the virus. This is important as inactivation treatments lead to epitope modifications ( Cruz et al., 2002 ). As the structural morphology of VLPs is similar to the virus, the conformational epitopes presented to the immune system are the same as for the native virus particles. The immune response/antibody reactivity in the case of VLPs is significantly improved as VLPs present conformation epitopes more similar to the native virus. VLPs also induce a strong B-cell response. For broader and more efficient protection, it is possible to adapt one or more antigens to the multimeric protein structure. Another advantage offered by VLPs is that they significantly reduce vaccine costs as these can elicit a protective response at lower doses of antigen.
Vaccines based on virus-like particles
The FDA has approved VLP-based vaccines for HBV and HPV. The HBV vaccine was approved in 1986 and the HPV one in 2006 ( Justin et al., 2011 ). To generate immunogenic VLPs, the S gene is cloned and expressed in a eukaryotic expression host such as yeast or mammalian cells (e.g., CHO cell line). The mammalian cell culture allows easy recovery because the cells are able to secrete the antigen HBsAg. The two companies producing CHO-based vaccines are the French-based Pasteur-Merieux Aventis (Gene Hevac B) and the Israeli-based SciGen (Sci-B-Vac). The Gene Hevac B vaccine contains the HBsAg S protein and M protein, whereas Sci-B-Vac contains the M and L proteins.
Human papilloma virus vaccine
Viruses of the Papillomaviridae family are known to induce lesions and warts and also cause cervical cancer. Fifteen strains of Papillomaviridae are known to cause cervical cancer. HPV-16 is considered a high-risk HPV type as the risk of cancer may be higher than for other high-risk HPV types. The two virally encoded proteins of HPV are L1 and L2. L1 is the main capsid protein that forms the outer shell of the virus. L2 is found in the interior of the viral particle and is less abundant. The recombinant L1 VLP is able to induce neutralizing antibodies in animals. Gardasil (the first HPV vaccine) was approved by the FDA in 2006. This vaccine is manufactured by Merck and Co., Inc. Ceravarix, another HPV vaccine (manufactured by Glaxo Smithkline), was approved by the FDA in 2009. It uses the Trichoplusia ni (Hi-5) insect cell line infected with L1 recombinant baculovirus ( Jiang et al., 1998 , Wang et al., 2000 ).
A number other VLP-based vaccines are in clinical trials. These include the anti-influenza A M2-HBcAg VLP vaccine ( Clarke et al., 1987 ), two antimalarial vaccine nicotine-Qβ VLPs ( Maurer et al., 2005 ), and an anti-AngIIQβ VLP. The VLP production in mammalian cell lines and Baculo cell lines of viruses infecting humans and other animals is summarized in Table 14.6 .
VLP production in mammalian and baculo cell lines of viruses infecting humans and other animals.
Virus | Expression system | Cells | Recombination protein | Reference |
---|---|---|---|---|
SIV | B/IC | Sf9 | Pr55 gag envelope protein | |
Ebola | Human cells | HEK293T | VP40 and glycoprotein | |
Rota virus | B/IC | Sf9 | VP2, VP6, VP7 | |
SARS-CoV | Baculovirus-insect cell system | Sf21 | E and M proteins | |
SARS | Insect cell culture system | Sf-9 | S protein, E protein, and M protein | |
Marburg and Ebola virus | Mammalian cell culture system | 293T cells | VP40 and glycoproteins | |
AAV | Insect cell culture system and mammalian cell culture system | Sf-9 and HEK 293 | VP1, VP2, and VP3 | |
HIV-1 | Baculovirus-insect cell expression system | Sf-21 | HIV gag proteins like GagTN and GagRT | Pillay et al. (2009) |
Influenza A | Baculovirus expression system | Sf-9 | H1N1 protein, M1 protein, etc. | Krammer et al. (2010) |
MERS-CoV | Recombinant baculoviruses (rBVs) | Sf9 | Spike (S), envelope (E), and membrane (M) protein | |
ZIKV | CHO | HEK293 | rH7 antigens |
AAV, adeno-associated virus; rBVs , recombinant baculoviruses; ZIKV , Zika virus.
Recombinant therapeutic proteins
Proteins play a major role in carrying out biochemical reactions, transporting small molecules within a cell or from one organ to another, formation of receptors and channels in membranes, and providing frameworks for scaffolding. The number of functionally distinct proteins in humans far exceeds the number of genes as a result of post-translational modifications. These modifications include glycosylation, phosphorylation, ubiquitination, nitrosylation, methylation, acetylation, and lipidation. The changes in protein structure as a result of mutation or other abnormalities often lead to a disease condition. Protein therapeutics offer tremendous opportunities for alleviating disease. The first therapeutic from recombinant mammalian cells was human tissue plasminogen, which obtained market approval in 1986. At present, 60%–70% of all the recombinant therapeutic proteins are produced in mammalian cells.
Main therapeutic proteins
The main therapeutic proteins can be divided into seven groups ( Walsh, 2003 ):
- 1. Cytokines
- 2. Hematopoietic growth factors
- 3. Growth factors
- 4. Hormones
- 5. Blood products
- 7. Antibodies
Most of the proteins have complex structures and undergo chemical modification to insure full biological activity. Protein post-translation modifications (PTM) can happen in several ways. The most widely recognized form of PTM is glycosylation, which involves extensive sequence processing and trimming in the Golgi apparatus and endoplasmic reticulum. Eukaryotic cells are capable of carrying out this type of modification and are thus preferred in biopharmaceutical processes. Hamster, baby hamster kidney (BHK), and CHO cells are often the host cells of choice as glycosylation patterns generated from these cells are more similar to human patterns. Table 14.7 lists various therapeutic proteins produced in animal cell lines.
Various therapeutic proteins produced in animal cell lines.
Cell line | Therapeutic protein | Potential application | Product name | Approval (FDA) |
---|---|---|---|---|
CHO | tPA | Acute myocardial infraction | Activase | 1987 |
BHK | Factor VIII | Hemophilia A | Kogenate FS | 1993 |
Sp2/0 | Immunoglobulin GI | Rheumatoid arthritis, Crohn’s disease | Remicade | 1998 |
BHK | Factor VIIa | Hemophilia A+B | Novo Seven | 1999 |
HEK-293 | Activated protein C | Severe sepsis | Xigris | 2001 |
Hybridoma CHO | Immunoglobulin G2a | Non-Hodgkin’s lymphoma | Bexxar | 2003 |
CHO | Immunoglobulin GI | Colorectal cancer | Avastin | 2004 |
CHO | Humanized IgG | Cancer | Perjeta | 2012 |
CHO | Humanized IgG1; DM1 | Cancer | Kadcyla | 2013 |
CHO | Humanized IgG4κ | Cancer | Keytruda | 2014 |
CHO | Human IgG4κ | Cancer | Opdivo | 2014 |
NS0 | Humanized IgG1 | Cancer | Empliciti | 2015 |
CHO | Human IgG1κ | Cancer | Darzalex | 2015 |
CHO | Humanized IgG1 Fab | Hemostasis | Praxbind | 2015 |
CHO | Human IgG1 | Cancer | Bavencio | 2017 |
CHO | Humanized IgG1/κ | Asthma | Fasenra | 2017 |
CHO | Human IgG1λ | Psoriasis | Tremfya | 2017 |
CHO | Glycosylated IgG1 | Multiple sclerosis | Ocrevus | 2017 |
CHO | Human recombinant IgG1 | Rheumatoid arthritis | Kevzara | 2017 |
Cytokines are proteins of the immune system that play a central role in immune response. Cytokines are produced as a result of immune stimulus by various white blood cells. Interferons (IFNs) were the first family of cytokines to be discovered and used as biopharmaceuticals.
Applications of interferons
IFNα is used for treatment of hepatitis, and more recently has been approved for leukemia and other types of cancers. IFNβ is used for treatment of multiple sclerosis and is marketed under the names Avonex, Belaseron, and Rebif. IFNγ is used for the treatment of chronic granulomatous disease. Interleukin is another kind of cytokine that helps regulate cell growth, differentiation, and motility and is used as a biopharmaceutical. The recombinant form of IL-2 is used for the treatment of renal cell carcinoma.
Growth factors
Growth factors are proteins that bind to receptors on the surface of cells to activate the cells for proliferation and or differentiation. The different types of growth factors are TGF, insulin-like growth factor, and (EGF. The primary sources of PDGF are platelets, endothelial cells, and the placenta. Two isoforms of this protein are present in the human body and both of these have one glycosylation site and three disulfide bonds. Examples of growth factors used as biopharmaceuticals are the following:
- 1. Osigraft/Eptotermin alfa (bone morphogenetic protein) is used for the treatment of tibia fractures, is grown commercially in CHO cells, and was first approved in 2001 in Europe.
- 2. InductOS/Dibotermin (bone morphogenetic protein) is used for tibia fractures and in spinal surgery; it is also commercially grown in CHO cells. This product was first approved in Europe in 2002.
Insulin, glucagon, gonadotropins, and growth hormones are the most well-known therapeutic hormones. The first biopharmaceuticals that obtained approval by regulatory agencies were insulin and recombinant human growth hormones. These were produced in microbial cells. The commercial recombinant forms of the gonadotropin family of hormones are Gonal-F, Luveris, Puregon, and Ovitrelle. All these are produced using CHO cells and are used for treating female infertility.
Therapeutic enzymes
A number of recombinant therapeutic enzymes are expressed in mammalian cells. Tissue plasminogen activator (tPA) is a thrombolytic agent involved in dissolving blood clots. Recombinant tPA is commercially is known as Alteplase and Tenectplase, which are used for the treatment of acute myocardial infraction.
Fabry disease, a genetic metabolic disorder, is characterized by a lack of enzyme α-galactosidase A. Fabrazyme (approved in 2001) is a recombinant α-galactosidase A and is produced by genetically modified CHO cells.
Blood coagulation factors
Hemophilia A is caused by the lack of blood-clotting factor VIII, hemophilia B is caused by deficiency of factor IX, and hemophilia C by lack of factor XI. Factor VIII and IX are proteins. The first recombinant factor VII products were Recombinate and Kogenate, which were expressed in CHO and BKH cells, respectively. Recombinant factor FIX is commercially sold as BeneFIX and is produced in recombinant CHO cells.
Therapeutic antibodies are used in the treatment of cancer, cardiovascular disease, infections, and autoimmune diseases. In 2004, the antibody Avasin (Bevacizeimab) was approved for the treatment of metastatic colorectal cancer. This antibody acts as an inhibitor of vascular endothelial growth factor. Zenapax, another commercially available antibody, is used during prophylaxis for preventing the rejection of transplanted organs. This is commercially grown in the NSO cell line and was approved for human use in 1997.
Gene therapy
Importance of cell culture in gene therapy.
Gene therapy involves the insertion, removal, or alteration of a therapeutic or working gene copy to cure a disease or defect or to slow the progression of a disease, thereby improving the quality of life. The human genome map was the first major step toward a new way of addressing human health and illness. Gene therapy holds great promise, however, the task of transferring genetic material into the cell remains an enormous technical challenge and requires ex vivo cell cultivation and adaptation from the lab to a clinically relevant state. The development of animal cell culture technology is imperative for advances in gene therapy.
Monogenic diseases caused by single gene defects (such as cystic fibrosis, hemophilia, muscular dystrophy, and sickle cell anemia) are the primary targets of human gene therapy.
The first step in gene therapy is to identify the faulty gene. This is followed by gene isolation and generation of a construct for correct expression. Integration of the gene followed by delivery of the genetic material in vivo or ex vivo is crucial to the success of gene therapy. In in vivo therapy, the genetic material is introduced directly into the individual at a specific site, and in ex vivo treatment, the target cells are treated outside the patient’s body. These cells are then expanded and transferred back to the individual at a specific site. The ex vivo technique involves gene therapy in the cultured cells, which are expanded and subsequently transferred to the targeted tissue.
Clinical correlation
A number of clinical studies and trials for gene therapy have already been approved and are being conducted worldwide. From 1989 up to the present, about 500 clinical studies have been reported; 70% of these studies are intended for cancer treatment.
The first product designed for gene therapy was Gendicine, a medication produced by Shenzhen Sibiono Genetech, China. Gendicine is used for head and neck carcinoma treatment. The tumor 4 suppressing gene p53 in recombinant adenovirus expresses protein p53, which leads to tumor control and elimination. SBN-cel is a cell line that was subcloned from the human embryonic kidney (HEK) cell line 293 and has been used for the production of Gendicine.
Biopesticides
In recent years, biopesticides have gained importance due to increased concerns about agrochemicals and their residues in the environment and food. Biopesticides provide an effective means for the control of insects and plant disease, and they are environmentally safe. The biological control of insect pests by another living organism (in order to suppress the use of pesticides) is an age-old practice. Presently, a number of biological controls are being used as biopesticides. With the high cost of chemical-based pesticides and the development of resistance to multiple chemical pesticides, baculoviruses are one of the most promising biocontrols for insect pests and have been increasingly used effectively against caterpillars worldwide. However, the major impediment in the development of baculoviruses as biopesticides is the high cost and small volumes of in vitro methods. Development of an in vitro production process for large quantities of baculoviruses at comparable costs to chemical pesticides will help provide insect control that is safe, efficacious, cost-effective, and environmentally safe.
Baculovirus production in animal cell culture
A number of factors are important for a successful commercial production of bioinsecticides:
- 1. Large-scale production of viruses at competitive costs.
- 2. Economic production of viruses (i.e., low cost for the media and running the culture).
- 3. Effective cell line with high virus per cell productivity.
- 4. With passage of the virus into cells, there is a loss of virulence and an increased risk of mutant formation; this should be avoided.
- 5. The quality of the polyhedral produced in the cell culture should be comparable to those obtained from caterpillars.
The insect baculovirus cell system offers a number of advantages. It produces recombinant proteins that are functional and are immunologically active, as it is able to make post-translational modifications. The recombinant system uses a powerful promoter polyhedron.
Cell lines for biopesticide production
The most commonly used cell lines in biopesticide production are the Sf21 and Sf9 cell lines, which are derived from ovarian tissues of the fall army worm ( Spodoptera frugiperda ). Sf9 cells show a faster growth rate and higher cell density than Sf21 cells and are preferred. High Five cell lines (designated BTI-Tn-5BI-4) established from Trichoplusia ni embryonic tissue are also being used.
Viral mutant formation in cell culture
The continuous culturing of cells for virus production leads to virus instability and the so-called passage effect. This can result in a decrease of virulence and polyhedral production and a variety of mutations. All these changes affect commercial production in vitro. Two types of mutations are commonly seen in continuous passaging of cell cultures for viral productions: (1) defective infective particles (DIPs) and (2) few polyhedral (Fp) mutations.
Fp mutations are characterized by (1) reduced polyhedral, (2) enhanced production of BV, and (3) lack of occluded virions in polyhedra. All these factors reduce the infectivity of the target pest.
Spontaneously generated Fp mutants have been reported in AcMNPV ( Autographa California nucleopolyhedroviruses) ( Wood, 1980 ), Galleria mellonella nucleopolyhedroviruses (GmMNPV) ( Fraser and Hink, 1982 ), and Helicoverpa armigera nucleopolyhedroviruses (HaSNPV) ( Chankraborty and Reid, 1999 ).
DIP mutations are the formation of DIPs. They occur due to serial passaging for long periods, which results in a decrease in the filtering of infectious virus. DIPs have been reported in a number of animal virus systems and in baculovirus systems. DIP formation can be avoided by low multiplicity of infection. This minimizes the probability of the defective virus entering the cell with an intact helper virion.
Monoclonal antibodies
The majority of antibodies available on the market today are produced in animal cell cultures ( Van Dijk and Van de Winkle, 2001 ). Animal cells are preferred because they are capable of glycosylation and structural conformation, which is essential for a drug to be productive. Hybridoma technology has been the most widely used method for small- and large-scale production of mABs. However, these antibodies have limited therapeutic applications since they produce an adverse immune response on repeated use.
A number of cell lines are now being used for the production of recombinant antibodies. The CHO lines are the most commonly used. Other cell lines used are marine myelomas NSO, Sp 2/0, HEK-93, and BHK.
A number of factors influence the production of mABs. For a high concentration of mAB production, the cell line should have high productivity. For high protein productivity, it is important that the selected cell line be productive in order to avoid large reaction volumes and the high cost of protein purification. Cell lines with the capacity to grow without anchorage offer an advantage in terms of scaling up the process; it is much simpler than with those designed for the growth culture of anchorage-dependent cells. Sp2/0 and NSO cell lines can grow naturally in suspension; other cells such as CHO and BHK can be easily adapted to this form of cultivation.
Stem cells are unspecified cells that have the potential to differentiate into other kinds of cells or tissues and become specialized cells. The two characteristics that define stem cells are their ability of self-regenerate and to differentiate into any other cells or tissues. These cells have the capability to renew themselves to form cells of more specialized function. In recent years, stem cell research has been hailed as a major breakthrough in the field of medicine. This property of turning a cell into any other specialized function cell has made researchers believe that stem cells could be utilized to make fully functional, healthy organs to replace damaged or diseased organs.
Culturing embryonic stem cells in the laboratory
Human embryonic stem cells (hESCs) are grown on nutrient broth. These cells are traditionally cultured on mouse embryonic fibroblast feeder layers, which allows continuous growth in an undifferentiated stage. The mouse cells at the bottom of the culture dish provide a sticky surface to which the cells can attach. In addition, the feeder cells release nutrients into the culture medium. Researchers have now devised animal-free culture systems for hESCs and have used human embryonic fibroblasts and adult fallopian tube epithelial cells as feeder layers (in addition to serum-free mediums).
More recently, methods to subculture embryonic cells without the feeder layer have been developed. Martigel from BD Biosciences has been used to coat the culture plate ( Hassan et al., 2012 ) for effective attachment and differentiation of both normal and transformed anchorage-dependent epithelioid and other cell types. This is a gelatinous protein mixture isolated from mouse tumor cells.
Microfluidics three-dimensional culture
A major milestone in the biological sciences was the establishment of the tissue culture technique that can both maintain and propagate the growth of living cells under sterile in vitro conditions. Traditional cell cultures, which are two-dimensional (2D), are grown as monolayer cultures on a flat and rigid surface. Since their development, several advancements have been made to improve cell culture media as well as the biological materials used for culturing. The improvements have proven valuable for cell-based study due to their amalgamation of various modern analytical techniques, such as fluorescence, electrochemistry, and mass spectroscopy. 2D cell culture does not provide an adequate in vivo environment, where other cells surround the cells in a three-dimensional (3D) ECM ( Edmondson et al., 2014 ). Cells under in vivo conditions both produce and continuously consume oxygen nutrients and other molecules, and such dynamic distributions are not mimicked in conventional 2D cell cultures. Moreover, 2D cell cultures fail to recapitulate the highly complex 3D environment, function, and physiology of living tissues, the multitudinous regulatory interactions from surrounding tissue cells, the ECM, and other systemic factors that lead to nonpredictive data of an in vivo response ( Li et al., 2012 ). The limitations of 2D cell culture systems have recently become more evident. Recent standard protocol advances in the fields of quantitative and system biology and imaging technology have allowed analysis of individual cells and observation of live individual cells growing in a natural physiological 3D environment. Cells cultured in a 3D model system more closely mimic in vivo conditions. Thus, unlike 2D cell cultures, which can sometimes cause misleading and nonpredictive data of in vivo responses, 3D systems are realistic for translating study findings. Compared to the 2D cell culture system, the 3D cell culture system provides a physiologically relevant and closer biomimetic environment, promotes better cell differentiation, and improves cell function ( Edmondson et al., 2014 ). The 3D culture system holds great promise for applications in various fields, such as cancer cell biology, stem cell research, drug discovery, and various cell-based analyses and devices. While this culturing model offers state-of-the-art technology for facilitating drug development and numerous other applications, several hurdles remain before a universal, standardized, and validated system can be established ( Sung et al., 2014 ). Recent developments in the transition from 2D to 3D cell cultures indicate promising applications for many industries; however, the cost of automation and easy-to-use readout systems are still key concerns.
The 3D cell culture system has provided a powerful tool that mimics a highly complex and dynamic in vivo environment, and it has gained greater momentum with the integration of microfluidic technology. Microfluidics is a technology characterized by the manipulation of fluids at the micron-scale for the improvement of diagnostics and cell culture research. It uses microfluidic devices to manipulate fluids in the small capillaries or microchannels. Microfluidics is a science of manipulating, mixing, monitoring, and analyzing minute volumes of fluids or gases on the surfaces of chips and microfluidic chips. This technology is ideal because it recreates the microenvironment of the vasculature and has become a powerful tool in cell culture research. It encompasses knowledge of the biological sciences, chemistry, physics, and engineering applications ( Xu and Attinger, 2008 ). The microfluidic 3D cell culture model also allows precise spatial control over the gradients and medium exchange. It not only mimics but also promotes several biologically relevant functions not seen in the 2D cell culture. Furthermore, it has been increasingly used to generate high-throughput cell culture models and has shown considerable promise for improving diagnostics and biological research ( El-Ali et al., 2006 ).
Notably, microfluidic cell cultures are potential candidates for next generation cell analysis systems. Several 3D-based cell culture approaches have been created to provide a better biomimetic microenvironment for cells than those of 2D cultures. In addition, crucial liquid handling steps, including cell loading, nutrient supply, and waste removal—under physiologically relevant conditions—can be performed with real-time microscopy ( Xu et al., 2014 ). Numerous microfluidic devices have been developed to not only provide nutrients and oxygen continuously for cell proliferation but also to investigate several characteristics of a dynamic 3D cell culture, such as differences in concentration, temperature gradients, and shear force conditions on cell transport and cultivation. Numerous microfluidic platforms for 3D cell culturing have been developed and based on the substrates used for microdevice fabrication, including glass/silicon-based, polymer-based, and paper-based platforms. Polydimethylsiloxane (PDMS)-based microdevices are the predominant form of microfluidic 3D cell culture systems because they are economical and allow permeability of O2, which is vital in cell proliferation. To provide an in vivo-like environment that resembles living tissues, several natural polymers, such as collagen, fibrin, and agarose, have been used to fabricate microfluidic devices ( Li et al., 2012 ).
Applications
Microfluidics technology has emerged as a viable and robust platform for tissue engineering—a multidisciplinary field aimed at replacing and repairing damaged and diseased tissues and/or organs and developing in vitro models to mimic physiological conditions. Successful clinical applications include the development of organ-on-a-chip technology—a microfluidic perfusion device for regenerative medicine—and a chip-based platform for the culture of cells and toxicological studies.
Organ-on-a-chip technology
Scientists currently rely on in vitro cell culture platforms and in vivo animal models to study biological processes and develop therapeutic strategies, although informative have significant shortcomings ( Ziółkowska et al., 2011 ). In vitro platforms may not simulate the intricate cell–cell and cell–matrix interactions that are vital to regulating cell behavior in vivo ( Guillouzo & Guguen-Guillouzo, 2008 ). Organ-on-a-chip devices could offer biological relevance and be a requisite for high-throughput applications. An organ-on-a-chip is a microfluidic cell culture device comprising a microchip with continuously perfused chambers that are infused with living cells that are arranged to mimic the 3D tissue microenvironment and physiology ( Ghaemmaghami et al., 2012 ). These chips have the potential to significantly impact drug discovery and toxicity testing ( Ghaemmaghami et al., 2012 ). The simplest functional unit of organ-on-a-chip devices consists of a single, perfused microfluidic chamber that is composed of a single type of cultured cell. These systems are utilized for studying organ-specific responses, chemical responses, such as drugs or toxins, and physical stimuli. In a complex system, two or more independently perfused parallel microchannels are connected by porous membranes to recreate interfaces between different tissues.
Tissue models on a chip
Numerous tissue models have been developed to mimic in vivo biological processes. On-chip tissue models include those for the liver, kidney, lungs, intestines, muscle, fat, and blood vessels as well as models of tumors.
Liver-on-a-chip
Various chemicals and drugs, when administered over a long period, result in adverse effects and acute liver toxicity, known as hepatotoxicity ( Gershell & Atkins, 2003 ). In vitro models used for identifying drug-induced liver toxicity have drastically limited utility. Therefore, efficient and reliable tools for testing liver toxicity are required. Microfluidics devices for liver tissue and cells that can maintain metabolic activity and can be used for drug discovery and toxicity studies have shown great potential for solving this problem.
Bioreactors with a perfused multiwell plate device were developed by Domansky et al. (2010) to recapitulate both the physiological and mechanical microenvironments of hepatocytes that can support both growth and functional integrity for up to 1 week. Khetani and Bhatia (2008) developed microscale cultures of human liver cells in a multiwell micropatterned co-culture system that can maintain phenotypic functions of liver cells for up to several weeks.
Tumor-on-a-chip
A significant challenge for cancer research is the early detection and development of in vitro strategies for studying the role of drug-carrier design in tumor transport and therapies for targeting rapidly dividing cancer cells while leaving normal, healthy cells untouched. The microfluidics tumor-on-a-chip platform can be used for detecting circulating tumor cells (CTCs) in blood flow, which may lead to early diagnosis of cancer ( Millner et al., 2013 ). A variety of designs for studying the microenvironment of microfluidic devices that culture solid and liquid tumors were reviewed by Young (2013) . Tatosian and Shuler (2009) developed a novel microfluidic system to study the multidrug resistance of cancer cells to chemotherapeutic combinations. Jang et al. (2011) fabricated a microfluidic device with an active injection system that produced 64 of 100 combinations of different chemical solutions at various concentrations and stored them in isolated chambers. To optimize system parameters for varied types of cancer cells while requiring minute amounts of reagents and cells, Jedrych et al. (2011) generated a microfluidics system for photodynamic therapy-based measurements. This system allows light-induced photosensitizers to be delivered to the carcinoma cells, which—on reaction with oxygen—produce a chemical toxin that is lethal to tumor cells.
World Wide Web resources
http://www.fda.gov/downloads/biologicsbloodvaccines/guidancecomplianceregulatoryinformation/guidances/vaccines/ucm202439.pdf
http://www.fda.gov/BiologicsBloodVaccines/Vaccines/ApprovedProducts/ucm205541.htm
The Food and Drug Administration (FDA or USFDA) protects and promotes public health through the regulation of all foods (except meats and poultry), the nation’s blood supply, and other biologics (such as vaccines and transplant tissues). Drugs must be tested, manufactured, and labeled according to FDA standards before they can be sold or prescribed.
http://www.promega.com/~/media/files/products%20and%20services/na/webinars/mechanism%20of%20toxicitywebinar2.pdf?la=en
Promega manufactures enzymes and other products for biotechnology and molecular biology.
http://www.who.int/biologicals/publications/trs/areas/vaccines/cells/WHO_TRS_878_A1Animalcells.pdf
The World Health Organization (WHO) is a specialized agency that is concerned with international public health. It is affiliated with the United Nations and headquartered in Geneva, Switzerland. WHO ensures that more people, especially those living in dire poverty, have access to equitable, affordable care, so that they can lead healthy, happy, and productive lives.
http://amgenscholars.com/images/uploads/contentImages/biotechnology-timeline.pdf
Amgen Scholars provides hundreds of undergraduate students with the opportunity to engage in a hands-on summer research experience at some of the world’s leading institutions.
http://monographs.iarc.fr/ENG/Monographs/vol90/mono90-6.pdf
The IARC monographs identify environmental factors that can increase the risk of human cancer. These include chemicals, complex mixtures, occupational exposures, physical agents, biological agents, and lifestyle factors.
http://www.iptonline.com/articles/public/IPTFIVE76NP.pdf
IPTonline publishes “The Pharmaceutical Technology Journal,” which is designed to provide information on the latest ideas, cutting-edge technologies, and innovations shaping the future of pharmaceutical research, development, and manufacturing.
http://www.aceabio.com/UserFiles/doc/literature/xcell_appnotes/RTCA_AppNote07_ACEA_LoRes.pdf
ACEA Biosciences, Inc. (ACEA) is a privately owned biotechnology company. ACEA’s mission is to transform cell-based assays by providing innovative and cutting-edge products and solutions to the research and drug discovery community.
- Aucoin M.G., Jacob D., Chahal P.S., Meghrous J., Bernier A., Kamen A.A. Virus-like particle and viral vector production using the baculovirus expression vector system/insect cell system. Methods Mol. Biol. 2007; 388 :281–296. [ PubMed ] [ Google Scholar ]
- Bhat S.M. Animal Cell Culture Concept and Application. Alpha Science International Limited; Oxford: 2011. [ Google Scholar ]
- Caplen H., Peters B.J., Bishop D.H.L. Mutagen-directed attenuation of Rift Valley fever virus as a method for vaccine development. J. Gen. Virol. 1985; 66 :2271–2277. [ PubMed ] [ Google Scholar ]
- Chakraborty S., Reid S. Serial passage of a Helicoverpa armigera nucleopolyhedrovirus in Helicoverpa zea cell cultures. J. Invertebr. Pathol. 1999; 73 :303–308. [ PubMed ] [ Google Scholar ]
- Chen T.H., Liu W.C., Chen I.C., Liu C.C., Huang M.H., Jan J.T. Recombinant hemagglutinin produced from Chinese Hamster Ovary (CHO) stable cell clones and a PELC/CpG combination adjuvant for H7N9 subunit vaccine development. Vaccine. 2019; 37 (47):6933–6941. [ PMC free article ] [ PubMed ] [ Google Scholar ]
- Cho M.H., Niles A., Huang R., Inglese J., Austin C.P., Riss T. A bioluminescent cytotoxicity assay for assessment of membrane integrity using a proteolytic biomarker. Toxicol. Vitro. 2008; 22 :1099–1106. [ PMC free article ] [ PubMed ] [ Google Scholar ]
- Clarke B.E., Newton S.E., Carroll A.R., Francis M.J., Appleyard G., Syred A.D. Improved immunogenicity of a peptide epitope after fusion to hepatitis B core protein. Nature. 1987; 330 :381–384. [ PubMed ] [ Google Scholar ]
- Cruz P.E., Maranga L., Carrondo M.J.T. Integrated process optimisation: lessons from retrovirus and virus like production. J. Biotechnol. 2002; 99 :199–214. [ PubMed ] [ Google Scholar ]
- Domansky K., Inman W., Serdy J., Dash A., Lim M.H., Griffith L.G. Perfused multiwell plate for 3D liver tissue engineering. Lab. a chip. 2010; 10 (1):51–58. [ PMC free article ] [ PubMed ] [ Google Scholar ]
- Eagle H. Amino acid metabolism in mammalian cell cultures. Science. 1959; 130 :432–437. [ PubMed ] [ Google Scholar ]
- Edmondson R., Broglie J.J., Adcock A.F., Yang L. Three-dimensional cell culture systems and their applications in drug discovery and cell-based biosensors. Assay. drug. Dev. Technol. 2014; 12 (4):207–218. [ PMC free article ] [ PubMed ] [ Google Scholar ]
- El-Ali J., Sorger P.K., Jensen K.F. Cells on chips. Nature. 2006; 442 (7101):403–411. FDA guidelines. ( http://www.fda.gov/downloads/biologicsbloodvaccines/guidancecomplianceregulatoryinformation/guidances/vaccines/ucm202439.pdf2012 . [ PubMed ] [ Google Scholar ]
- Fraser M.J., Hink W.F. The isolation and characterization of the MP and FP plaque variants of Galleria mellonella nuclear polyhedrosis virus. Virology. 1982; 117 :366–378. [ PubMed ] [ Google Scholar ]
- Freshney R.I. Culture of Animal Cells: A Manual of Basic Technique. 3rd ed. Wiley-Liss Inc; New York: 1994. [ Google Scholar ]
- Freshney R.I. Culture of Animal Cells: A Manual of Basic Technique and Specialized Applications. Wiley, John & Sons, Inc; New Jersey: 2010. [ Google Scholar ]
- Freshney R.I. Culture of Animal Cells: A Manual of Basic Technique and Specialized Applications. Wiley, John & Sons, Inc; New Jersey: 2011. [ Google Scholar ]
- Gershell L.J., Atkins J.H. A brief history of novel drug discovery technologies. Nat. Rev. Drug. Discovery. 2003; 2 (4):321–327. [ PubMed ] [ Google Scholar ]
- Ghaemmaghami A.M., Hancock M.J., Harrington H., Kaji H., Khademhosseini A. Biomimetic tissues on a chip for drug discovery. Drug. Discov. Today. 2012; 17 (3):173–181. [ PMC free article ] [ PubMed ] [ Google Scholar ]
- Guillouzo A., Guguen-Guillouzo C. Evolving concepts in liver tissue modeling and implications for in vitro toxicology. Expert. Opin. drug. Metab. Toxicol. 2008; 4 (10):1279–1294. [ PubMed ] [ Google Scholar ]
- Hassan F., Ren D., Zhang W., Gu X.-X. Role of c-Jun N-terminal protein kinase 1/2 (JNK1/2) in macrophage-mediated MMP-9 production in response to Moraxella catarrhalis lipooligosaccharide (LOS) PLoS ONE. 2012; 7 :e37912. [ PMC free article ] [ PubMed ] [ Google Scholar ]
- Hayflick L., Moorhead P.S. The serial cultivation of human diploid cell strains. Exp. Cell Res. 1961; 3 :585–621. [ PubMed ] [ Google Scholar ]
- Ho Y., Lin P.H., Liu C.Y., Lee S.P., Chao Y.C. Assembly of human severe acute respiratory syndrome coronavirus-like particles. Biochemical and biophysical research communications. 2004; 318 (4):833–838. [ PMC free article ] [ PubMed ] [ Google Scholar ]
- Jang Y.H., Hancock M.J., Kim S.B., Selimović Š., Sim W.Y., Bae H. An integrated microfluidic device for two-dimensional combinatorial dilution. Lab. a Chip. 2011; 11 (19):3277–3286. [ PMC free article ] [ PubMed ] [ Google Scholar ]
- Jedrych E., Pawlicka Z., Chudy M., Dybko A., Brzozka Z. Evaluation of photodynamic therapy (PDT) procedures using microfluidic system. Analytica Chim. acta. 2011; 683 (2):149–155. [ PubMed ] [ Google Scholar ]
- Jeoung H.Y., Lee W.H., Jeong W., Shin B.H., Choi H.W., Lee H.S. Immunogenicity and safety of the virus-like particle of the porcine encephalomyocarditis virus in pig. Virology J. 2011; 8 :170. [ PMC free article ] [ PubMed ] [ Google Scholar ]
- Jiang B., Barniak V., Smith R.P., Sharma R., Corsaro B., Hu B. Synthesis of rotavirus-like particles in insect cells: comparative and quantitative analysis. Biotechnol. Bioeng. 1998; 60 :369–374. [ PubMed ] [ Google Scholar ]
- Justin C., Masum H., Perampaladas K., Heys J., Singer P.A. Indian vaccine innovation: the case of Shantha Biotechnics. Globalization Health. 2011; 7 :9. [ PMC free article ] [ PubMed ] [ Google Scholar ]
- Khetani S.R., Bhatia S.N. Microscale culture of human liver cells for drug development. Nat. Biotechnol. 2008; 26 (1):120–126. [ PubMed ] [ Google Scholar ]
- Li, X.J., Valadez, A.V., Zuo, P., & Nie, Z. (2012). Microfluidic 3D cell culture: potential application for tissue-based bioassays. [ PMC free article ] [ PubMed ]
- Licata J.M., Johnson R.F., Han Z., Harty R.N. Contribution of Ebola virus glycoprotein, nucleoprotein, and VP24 to budding of VP40 virus-like particles. Journal of virology. 2004; 78 (14):7344–7351. [ PMC free article ] [ PubMed ] [ Google Scholar ]
- Maurer P., Jennings G.T., Willers J., Rohner F., Lindman Y., Roubicek K. A therapeutic vaccine for nicotine dependence: preclinical efficacy, and phase I safety and immunogenicity. Eur. J. Immunology. 2005; 35 :2031–2040. [ PubMed ] [ Google Scholar ]
- Mello I.M.V.G., Meneghesso da Conceicao M., Jorge S.A.C., Cruz P.E., Alves P.M.M., Carrondo M.J.T. Viral vaccines: concepts, principles, and bioprocesses. In: Castilho L.R., Moraes A.M., Augusto E.F.P., editors. Animal Cell Technology: From Biopharmaceuticals to Gene Therapy. Taylor & Francis Group; New York: 2008. pp. 435–458. [ Google Scholar ]
- Millner L.M., Linder M.W., Valdes R. Circulating tumor cells: a review of present methods and the need to identify heterogeneous phenotypes. Ann. Clin. Lab. Sci. 2013; 43 (3):295–304. [ PMC free article ] [ PubMed ] [ Google Scholar ]
- Moore A., Donahue C.J., Hooley J., Stocks D.L., Bauer K.D., Mather J.P. Apoptosis in CHO cell batch cultures: examination by flow cytometry. Cytotechnology. 1995; 17 :1–11. [ PubMed ] [ Google Scholar ]
- Mortola E., Roy P. Efficient assembly and release of SARS coronavirus-like particles by a heterologous expression system. FEBS Lett. 2004; 576 :174–178. [ PMC free article ] [ PubMed ] [ Google Scholar ]
- Parez N., Garbarg-Chenon A., Fourgeux C., Le Deist F., Servant-Delmas A., Charpilienne A. The VP6 protein of rotavirus interacts with a large fraction of human naive B cells via surface immunoglobulins. Journal of virology. 2004; 78 (22):12489–12496. [ PMC free article ] [ PubMed ] [ Google Scholar ]
- Smithburn K.C. Rift Valley fever: the neurotropic adaption of virus and experimental use of this modified virus as a vaccine. Br. J. Exp. Pathol. 1949; 30 :1–16. [ PMC free article ] [ PubMed ] [ Google Scholar ]
- Stacey G.N., Davis J. Medicines from Animal Cell Culture. John Wiley & Sons; Chichester: 2007. [ Google Scholar ]
- Sung J.H., Srinivasan B., Esch M.B., McLamb W.T., Bernabini C., Shuler M.L. Using physiologically-based pharmacokinetic-guided “body-on-a-chip” systems to predict mammalian response to drug and chemical exposure. Exp. Biol. Med. 2014 1535370214529397. [ PMC free article ] [ PubMed ] [ Google Scholar ]
- Swenson D.L., Warfield K.L., Negley D.L., Schmaljohn A., Aman M.J., Bavari S. Virus-like particles exhibit potential as a pan-filovirus vaccine for both Ebola and Marburg viral infections. Vaccine. 2005; 23 (23):3033–3042. [ PubMed ] [ Google Scholar ]
- Tatosian D.A., Shuler M.L. A novel system for evaluation of drug mixtures for potential efficacy in treating multidrug resistant cancers. Biotechnol. Bioeng. 2009; 103 (1):187–198. [ PubMed ] [ Google Scholar ]
- van Dijk M.A., van de Winkel J.G.J. Human antibodies as next generation therapeutics. Curr. Opin. Chem. Biol. 2001; 5 :368–374. [ PubMed ] [ Google Scholar ]
- Walsh G. Biopharmaceuticals – Biochemistry and Biotechnology. 2nd ed. John Wiley and Sons; Chichester: 2003. [ Google Scholar ]
- Wang C., Zheng X., Gai W., Zhao Y., Wang H. MERS-CoV virus-like particles produced in insect cells induce specific humoural and cellular imminity in rhesus macaques. Oncotarget. 2017; 8 (8):12686. [ PMC free article ] [ PubMed ] [ Google Scholar ]
- Wang M.Y., Kuo Y.Y., Lee M.S., Doong S.R., Ho J.Y., Lee L.H. Self-assembly of the infectious bursal disease virus capsid protein, rVP2, expressed in insect cells and purification of immunogenic chimeric rVP2H particles by immobilized metal-ion affinity chromatography. Biotechnol. Bioeng. 2000; 67 :104–111. [ PubMed ] [ Google Scholar ]
- Wood H.A. Isolation and replication of an occlusion body-deficient mutant of the Autographa californica nuclear polyhedrosis virus. Virology. 1980; 105 :338–344. [ PubMed ] [ Google Scholar ]
- Xu J., Attinger D. Drop on demand in a microfluidic chip. J. Micromech. Microeng. 2008; 18 (6):065020. [ Google Scholar ]
- Xu X., Farach-Carson M.C., Jia X. Three-dimensional in vitro tumor models for cancer research and drug evaluation. Biotechnol. Adv. 2014; 32 (7):1256–1268. [ PMC free article ] [ PubMed ] [ Google Scholar ]
- Yamshcikov G.V., Ritter G.D., Vey M., Compans R.W. Assembly of SIV virus-like particles containing envelope proteins using a baculovirus expression system. Virology. 1995; 214 (1):50–58. [ PubMed ] [ Google Scholar ]
- Young E.W. Cells, tissues, and organs on chips: challenges and opportunities for the cancer tumor microenvironment. Integr. Biol. 2013; 5 (9):1096–1109. [ PubMed ] [ Google Scholar ]
- Ziółkowska K., Kwapiszewski R., Brzózka Z. Microfluidic devices as tools for mimicking the in vivo environment. N. J. Chem. 2011; 35 (5):979–990. [ Google Scholar ]
Further reading
- Castilho L.R., Moraes A.M., Augusto E.F.P. Animal Cell Technology. Taylor & Francis Group; New York: 2008. From Biopharmaceuticals to Gene Therapy. [ Google Scholar ]
- Freshney R.I. Culture of Animal Cells: A Manual of Basic Techniques and Specialized Applications. 7 th Edition. Wiley Blackwell; USA: 2015. [ Google Scholar ]
- Kolesnikova S., Moiseeva I. Prospects for the use of animal cell cultures in screening of pharmaceutical substances. J. Phys.: Conf. Ser. 2017; 784 :012028. doi: 10.1088/1742-6596/784/1/012028. [ CrossRef ] [ Google Scholar ]
- Luo Tao. Microfluidic single-cell manipulation and analysis: methods and applications. Micromachines. 2019; 10 (2):104. [ PMC free article ] [ PubMed ] [ Google Scholar ]
- Naskalska A., Dabrowska A., Szczepanski A., Milewska A., Jasik K.P., Pyrc K. Membrane protein of human coronavirus nl63 is responsible for interaction with the adhesion receptor. J Virology. 2019; 93 (19):e00355–19. [ PMC free article ] [ PubMed ] [ Google Scholar ]
- Polat A.N., Özlü N. Towards single-cell LC-MS phosphoproteomics. Analyst. 2014; 139 :4733–4749. [ PubMed ] [ Google Scholar ]
- Stecey G.N., Davis J. Medicines from Animal Cell Culture. John Wiley & Sons; Chichester : 2007. [ Google Scholar ]
- Verma A.S., Singh A. Animal Biotechnology: Models in Discovery and Translation. 1st Edition. Acadamic Press; USA: 2014. [ Google Scholar ]
- Yu F., Hunziker W., Choudhury D. Engineering microfluidic organoid-on-a-chip platforms. Micromachines. 2019; 10 (3):165. [ PMC free article ] [ PubMed ] [ Google Scholar ]
Abbreviations
Long answer questions.
- 1. What are the components of serum and how do they help the cell culture?
- 2. What is the role of media in animal cell culture?
- 3. What are the advantages and limitations of animal tissue culture?
- 4. How can cell viability and cytotoxicity be tested in cell culture?
- 5. What is the role of cell culture in gene therapy and viral vaccines?
- 6. How can microfluidics revolutionize animal tissue culture?
Short answer questions
- 1. What is the Hayflick effect?
- 2. What is the source of cells for primary monolayer cell culture?
- 3. Serum is one of the basic components of cell culture media (true/false)?
- 4. What was the first recombinant human protein?
- 5. What are the different phases of the growth curve?
- 6. Is the VLP-based HPV vaccine approved by the FDA?
Answers to short answer questions
- 1. Limited replication capacity of cells in culture medium.
- 2. Organ/tissue of live animal.
- 4. Somatostatin.
- 5. Lag phase, log phase, and plateau phase.
- 6. Yes, Gardasil (the first HPV vaccine) was approved by the FDA in 2006.
Yes/no type questions
- 1. Are cells obtained directly from organs and tissues in primary cell culture?
- 2. Is secondary culture used for studying transformed cells?
- 3. Is identity testing a way to determine purity of culture?
- 4. Is IFN-α used for the treatment of multiple sclerosis?
- 5. Is Bevacizumab approved for the treatment of colorectal cancer?
- 6. Does passage effect leads to an increase in the virulence of cultured viruses?
- 7. Do stem cells can not differentiate into other kinds of cells?
- 8. Microfluidic devices provide nutrients and oxygen for cell proliferation.
- 9. Living cells are used in organ-on-a-chip microfluidic cell culture.
- 10. Can embryonic cells be cultured without any feeder layer?
Answers to yes/no type questions
- 1. Yes—Mechanical, chemical, or enzymatic disintegration of tissues and organs is required in primary cell culture.
- 2. Yes—Secondary cultures are used in the study of transformed cells as these cultures maintain their cellular characteristics.
- 3. No—For testing the purity, one should use fluorescent staining PCR or ELISA.
- 4. No—IFN-β is used in the treatment of multiple sclerosis.
- 5. Yes—It is an inhibitor of vascular endothelial growth factor.
- 6. No—Passage effect leads to viral instability.
- 7. No—Stem cells can differentiate into other kind of cell types.
- 8. Yes—Microfluidic devices also help in investigating characteristics of 3D cell culture.
- 9. Yes—Chambers of organ-on-a-chip devices are continuously infused with living cells.
- 10. Yes—Martigel from BD biosciences can be used to coat the culture plate.
- Open access
- Published: 21 April 2022
Advancing genome editing to improve the sustainability and resiliency of animal agriculture
- Diane Wray-Cahen ORCID: orcid.org/0000-0002-2123-2711 1 ,
- Anastasia Bodnar ORCID: orcid.org/0000-0002-3320-825X 2 ,
- Caird Rexroad III ORCID: orcid.org/0000-0002-3683-6147 3 ,
- Frank Siewerdt ORCID: orcid.org/0000-0003-2884-4320 4 &
- Dan Kovich ORCID: orcid.org/0000-0001-6585-913X 5
CABI Agriculture and Bioscience volume 3 , Article number: 21 ( 2022 ) Cite this article
8333 Accesses
10 Citations
87 Altmetric
Metrics details
Animal agriculture faces unprecedented challenges, including the need to increase productivity to meet increasing demands for high quality protein while combating increasing pest and disease pressures, improving animal welfare, adapting to a changing climate, and reducing the environmental impact of animal agriculture. Genome editing, in concert with other existing technologies, has the potential to accelerate these efforts. The U.S. Department of Agriculture (USDA) supports research focused on delivering scientific solutions to these national and global agricultural challenges and transferring these solutions to farmers. Genome editing, along with a broad range of other tools, provides an opportunity for scientists, breeders, farmers, and ranchers to meet these challenges and provides additional benefits for society, including healthier and more resilient livestock, while reducing agriculture’s impact on the environment. Farmers and ranchers need a full toolbox of existing and innovative options. However, they will not be able to access these tools unless flexible approaches are in place that encourage innovation and allow safe innovations to be used on farms. Genome editing can help us achieve these goals only if global regulatory and policy approaches allow their use in agricultural breeding programs and deployment to farms. The global regulatory landscape for products of genome editing is rapidly evolving, with an increasing number of countries focusing more on characteristics of products and whether they could be achieved by conventional breeding, rather than the technologies used to create them. The livelihoods of people along the agricultural value chain depend upon countries’ regulatory and policy choices; regulatory approaches and how they are applied have a dramatic impact in determining what products are developed and who can afford to use these new biotechnologies. We need to step forward and continue the momentum towards regulatory approaches that encourage innovation to ensure continued access to a safe, abundant, and affordable food supply for future generations.
Introduction
Animal agriculture must be increasingly resilient and adaptable in order to support global food security and to protect and improve human health. Farmers need tools to do more with less: to increase agricultural production while fighting emerging disease threats and the effects of climate change while conserving and safeguarding rapidly diminishing natural resources. Meeting these challenges will require investments in research and timely deployment of discovered solutions. Genome editing, while not a panacea, presents a significant opportunity to improve animal health, welfare, and production efficiency; improve human nutrition; and address the causes and consequences of climate change.
A variety of traits for animals are being developed using genome editing and there is potential for many more (van Eenennaam 2017a ; Karavolias et al. 2021 ). Some traits address animal disease or pests and could reduce need for antibiotics or insecticides. Genome editing could also be used to create healthier and safer food products, or to improve animal welfare. Other traits aim to reduce the environmental footprint of animal agriculture or make animals more resilient to the effects of climate change. The agricultural applications of genome editing are many, but whether these opportunities are realized depends on (1) sufficient investments in research; (2) consumer and market acceptance; and (3) regulatory policy approaches that allow for use of genome editing in agricultural breeding programs.
The U.S. Department of Agriculture (USDA) has more than a century of experience in improving, protecting, and promoting food safety, and decades of experience in regulating products of biotechnology. Across its 29 agencies and offices there is tremendous expertise in animal production, breeding, and health as well as a long history of transferring needed solutions into the hands of farmers. USDA is committed to transforming America’s food system by building more resilient local and regional food systems and fairer markets for all producers, ensuring access to healthy and nutritious food in all communities, and growing new markets and streams of sustainable income for farmers and ranchers using climate-smart agricultural practices.
The USDA Agricultural Research Service (ARS) was founded to conduct intramural research to develop and transfer solutions to agricultural problems of high national priority (USDA-ARS 2021 ). The USDA National Institute of Food and Agriculture (NIFA) was established to fund extramural research on innovative solutions to issues related to agriculture, food, the environment, and communities (USDA-NIFA 2021b ). Together, these agencies address key national and global challenges. To be successful, the solutions developed through USDA-supported research must be made available to farmers.
The first genetically engineered animal was produced before the first genetically engineered plant, but the paths for introduction into production have been quite different. Even though animal scientists identified many promising traits over the years, including many that were introduced via genetic engineering, animal producers have so far been unable to reap the benefits of biotechnology. Just as it is crucial for builders and construction engineers to have the best tools available for the task at hand, farmers need access to the full range of tools to address agricultural challenges. Animal scientists and breeders, now more than ever, need genome editing tools to create innovative solutions to the threats facing animal agriculture. Genome editing presents hope for the future, but only if the necessary research investment is paired with policies that enable transfer of these innovations from labs to barns.
Biotechnology terminology
Agricultural biotechnology refers to a range of tools other than conventional breeding that can be used to change the genetic makeup of an organism (see Fig. 1 ). Biotechnology tools include genetic engineering and genome editing.
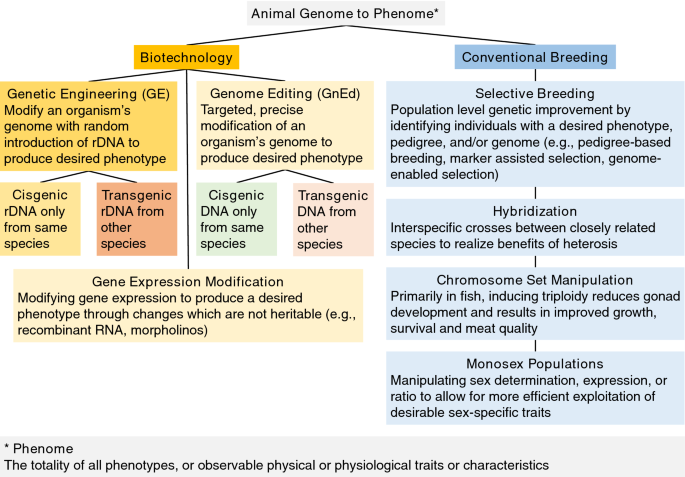
Definitions for and relationships between conventional and biotechnology tools used in animal breeding. These definitions may differ from colloquial use of the terms, and from how countries define these terms under their biotechnology regulations
Genetic engineering (GE) refers to the insertion of specific genes or gene variants at a random location in the genome using recombinant DNA (rDNA) techniques. Typically, an entire gene (promoter, coding sequence, and terminator) is inserted. Genetically engineered organisms are often colloquially referred to as GMOs or genetically modified organisms.
Genome editing (GnEd) refers to editing a genome at a precise location with enzymes called nucleases, using an organism’s own natural DNA repair mechanisms. Because of its precision, GnEd reduces the time and cost and increases the efficiency needed to develop new products. GnEd allows for a wider variety of outcomes, including changes that could have been created with breeding, such as deletions and substitutions.
Both GnEd and GE can be used to develop organisms with genetic material either from sexually compatible relatives ( cisgenic ) or from other species ( transgenic ) (see Fig. 2 ).
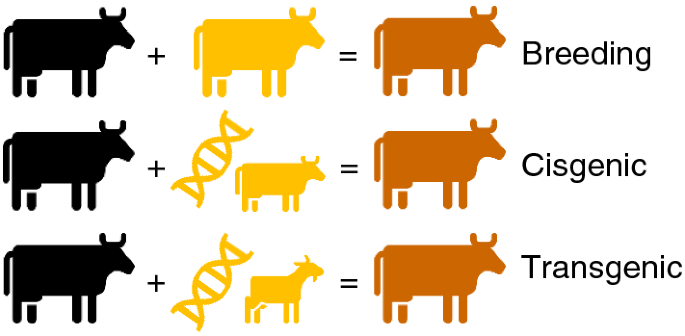
Hypothetical illustrations show animals developed with: conventional breeding where sexually compatible species are crossed using natural mating or assisted reproductive technologies, such as artificial insemination (top); “cisgenic” DNA sequences where the introduced sequence is from a compatible species and could have been introduced via conventional breeding (middle); “transgenic” DNA sequences where the introduced sequence is from a different species and could not have been introduced via conventional breeding (bottom)
Role of biotechnology in animal genetic improvement
Conventional breeding programs are the backbone of animal genetic improvement, securing incremental, cumulative, and permanent genetic gains. Conventional breeding methods include selective breeding, hybridization, and chromosome set manipulation (described in Fig. 2 ), as well as assisted reproductive techniques such as cloning, embryo transfer, and artificial insemination. These are all used to accelerate and/or amplify the rate of genetic gain in animal breeding programs. Biotechnologies, including GnEd, allow modification of phenotypes in ways that can reduce the time and cost to accomplish breeding goals. Biotechnologies can be combined with conventional breeding methods and can also be used to introduce traits that are not available via conventional breeding.
Advances in genome sequencing have led to better understanding of the fundamental biology underlying economic traits (Rexroad et al. 2019 ) and prediction of phenotypes associated with yield, production efficiency, animal health, well-being, and product quality. High-density sequencing in multiple livestock species has provided baseline information on genes, genome architecture, and genetic diversity. Public and private breeding programs can now rely on this information for decisions involving traits that are expensive or difficult to measure, typically measured postmortem, or expressed in only one sex. By combining this new resource with classical breeding programs, it is possible to accelerate rates of genetic progress in livestock, including shortening of the generation interval (Kasinathan et al. 2015 ), which brings particular benefit in dairy cattle and other animals with long generation times. Moreover, genomic information offers useful insights for improvement opportunities through GnEd.
Introducing desirable traits from beef cattle into dairy cattle or from laying hens into broilers can be accomplished by using GnEd without severe setbacks in important traits, such as milk production in dairy cattle or growth rates in broilers, that occur during introgression in conventional breeding programs. For animal welfare traits, GnEd offers novel solutions to address critical aspects of animal husbandry. While conventional breeding programs have documented progress in some animal welfare traits (Pryce and de Haas 2017 ; Siegel et al. 2019 ), combining GnEd with conventional genetic selection could speed genetic progress, with simultaneous advances in animal welfare and productivity. GnEd could also provide rapid animal welfare solutions to existing animal husbandry practices that have not been achieved with conventional breeding, such as dehorning in cattle, debeaking in poultry, castrating pigs and sheep, and culling of day-old male chicks in laying hen operations.
USDA’s role in animal genetic improvement
Animal genetic improvement programs require highly specialized genetics expertise as well as large investments of time and financial and animal resources. USDA’s role has been to support animal genetics policy, education, workforce development, and both intramural and extramural research for new technologies that produce animals with superior performance. To prioritize traits for genetic improvement, USDA regularly convenes government, university, and private sector animal breeders. Economically important traits are often species-specific, but generally are associated with a desire to (1) increase yields; (2) improve production efficiency, such as improved feed conversion ratios; (3) reduce on-farm losses to pests and pathogens; (4) improve animal well-being, including increased stress tolerance; (5) improve the sustainability of production or adaptability to climate change; (6) maintain or improve product quality; (7) increase food safety; and (8) maintain population genetic diversity.
Once priorities are determined, breeders develop approaches that will result in the desired genetic gains. For traits that can be directly and reliably measured in breeding populations, breeders may choose conventional quantitative genetic approaches. USDA-supported animal breeders have clearly demonstrated the benefits of these approaches for farmers and consumers over the last century, including enhancements obtained in the animals listed below. Each of these new animals developed via conventional breeding methods were transferred from USDA or university research stations to farmers, either directly or through a partnership with a private company.
George Washington is credited with developing mule breeding in the United States (Babb 2021 ). Mules are produced by crossing a male donkey and a female horse to produce offspring with optimal combined characteristics for labor compared to the parental species. In 1923, USDA published information on mules and mule breeding (Williams 1923 ).
The Beltsville Small White Turkey was developed by USDA researchers who began a breeding program in 1937 to create smaller turkeys better suited to meet consumer demands for a family sized turkey by crossing five genetic lines (Livestock Conservancy 2021 ).
Brangus cattle (3/8 Brahman and 5/8 Angus) were developed at the USDA Experiment Station in Jeanerette, Louisiana, to combine the heat and humidity tolerance of the Brahman and carcass quality of the Angus (GoBrangus 2008 ).
Targhee sheep were developed at the USDA Experiment Station in Dubois, Idaho, to meet producers’ need for a dual-purpose sheep with improved meat and wool (Taylor 2018 ).
Selective breeding of poultry began in the 1940s, which along with improved nutrition significantly shortened production times, improved feed conversion ratios, increased egg production, and increased both bird and egg size (Hunton 2006 ).
Hybrid catfish, a cross between male blue and female channel catfish, have better growth, higher survival, and better yield than purebred lines (Walker 2015 ). Developed with USDA funding, these hybrid catfish are now raised by more than 50% of the industry.
A research partnership between USDA-ARS and the Maine Aquaculture Association collects Atlantic salmon phenotypic information from commercial net pen production for use in genome-enabled breeding, with the goal of equitable germplasm distribution across the industry (USDA-ARS 2018 , 2019 ).
In 2008, USDA published the “Blueprint for USDA Efforts in Agricultural Animal Genomics 2008–2017” for animal genome research that prioritized basic and applied animal genomics research in 13 species, aiming to develop and implement new animal breeding tools and resources (Green et al. 2007 ). Between 2008 and 2017, ARS and NIFA spent ~ $500 M on research projects aiming to develop genome sequences, identify genetic variation, map genomes, characterize genome biology, and implement genomics into selective breeding strategies. Examples include:
Dairy cattle breeding, with centuries of gains from conventional selection, employed genomic selection to double rates of predicted gain, decrease generation interval, increase selection accuracy, reduce costs for progeny testing, and facilitate removal of recessive lethal alleles (Wiggans et al. 2017 ).
In beef cattle, genomic selection in Angus increased accuracy of genetic prediction for young animals, especially for traits with limited phenotypic information such as carcass traits, feed intake, and mature cow size (Moser et al. 2019 ), and assisted with management of lethal recessive alleles (Upperman et al. 2019 ).
In rainbow trout, genomic selection doubled selection accuracy for disease resistance (Vallejo et al. 2017 ).
In catfish, genomic selection increased predictive ability 28% for harvest weight and 36% for residual carcass weight (Garcia et al. 2018 ).
In each of these cases, the research results and new breeds were incorporated into commercial breeding programs. USDA updated and expanded the blueprint in 2018 by publishing “Genome to Phenome: Improving Animal Health, Production, and well-Being—A New USDA Blueprint for Animal Genome Research 2018–2027” that acknowledged successful applications of animal genome research, reflected advancements in genome technologies and expansion of applications of genomic information, and prioritized research activities in all agricultural animals (Rexroad et al. 2019 ). This updated blueprint added new goals, including enhanced use of genome editing and other biotechnologies, and preservation of genetic diversity.
USDA support for biotechnology
Support of agricultural research “with emphasis on biotechnology” became a priority beginning with the 1985 Farm Bill (U.S. House 1985 ), including “the effective transfer of new technologies, including biotechnology, to the farming community.” That same year, researchers at the USDA Beltsville Agricultural Research Center created the first genetically engineered livestock, a pig with a transgenic rDNA growth hormone gene inserted (Hammer et al. 1985 ). USDA-funded researchers and their colleagues around the world then began to develop transgenic methods for genetic improvement of pigs, sheep, and cattle in the 1980s and 1990s. The process of creating transgenic animals was inefficient and expensive (estimated to be $60,000 to $300,000, depending on the species) but with increases in efficiencies, there was an expectation that transgenic animals would be used in livestock production (Wall et al. 1997b ). Subsequently, the breakthrough development of mammalian cloning techniques using somatic cell nuclear transfer (Campbell et al. 1996 ) greatly improved the efficiency of rDNA technologies in livestock.
Agricultural biotechnology research, along with agricultural genome research, was among the priority mission areas listed in the Agricultural Research, Extension, and Education Reform Act of 1998 (U.S. House 1998 ). As with conventional breeding, USDA-funded researchers sought to apply biotechnology to further understand gene function and to obtain desired phenotypes (Murray and Maga 2016 ). Transgenic technologies, inserting a rDNA construct from non-host genome into a host embryo to obtain a desired phenotype, initially targeted enhanced production or disease resistance (Wall et al. 1997a ; Cao et al. 2015 ; Pursel and Rexroad 1993 ). Many agriculture-focused GE animals were developed with public funds in the United States and in other countries (van Eenennaam 2017a ; Wheeler 2013 ). Many of these animals had the potential to address issues of concern for both farmers and consumers but became lost opportunities for animal agriculture, as none were able to complete the difficult, expensive and, at the time, uncertain regulatory path to commercialization. Some examples include:
Mastitis resistant dairy cattle (Wall et al. 2005 ) and leaner more efficient pigs (Pursel et al. 2004 ) developed at USDA Beltsville Agricultural Research Center;
Suppression of prion protein in cattle (Golding et al. 2006 ; Richt et al. 2007 ) to create animals resistant to bovine spongiform encephalopathy (BSE);
Pigs and goats expressing factors in their milk with the potential to improve baby pig survival or enhance human health and nutrition (Bleck et al. 1998 ; Maga et al. 2006 ); and
Disease resistance in catfish (Dunham et al. 2002 ).
Since its inception in 2008, NIFA, USDA’s extramural research organization, has supported research covering several aspects of biotechnology through competitive and capacity-building programs. The 2008 Farm Bill (U.S. House 2008 ) established and set priorities for the Agriculture and Food Research Initiative (AFRI; USDA-NIFA 2021a ), a NIFA-administered competitive grants program. For Animal Health and Production and Animal Products, the priorities specifically included “animal biotechnology” and “identification of genes responsible for improved production traits and resistance to disease.” The expectation at that time was that solutions resulting from animal biotechnology research would be transferred to farmers as both the USDA-Animal and Plant Health Inspection Service (APHIS) and U.S. Department of Health and Human Services (HHS) Food and Drug Administration (FDA) were in the process of developing regulatory approaches for animals developed via biotechnology. However, when there did not appear to be a viable path to commercialize genetically engineered animals for use on farms, funding for agricultural biotechnology research declined. By 2014, no genetically engineered animals had been approved for food use, and animal biotechnology was no longer listed as a Farm Bill priority (U.S. House 2014 ). Creation of transgenic animals using rDNA and livestock cloning were early technologies that, despite low efficiencies and some scientific uncertainties, helped pave the road for newer methods, such as GnEd, that provide high technical precision and control over the final product.
USDA has long supported research to address priority issues in animal agriculture with a goal to develop solutions that can be delivered to farmers in a timely manner. The increased precision of GnEd techniques makes it easier to introduce genetic changes. They are also considerably less expensive to use than the rDNA technologies that preceded them and research using GnEd is being carried out around the world, including in developing countries (Tan et al. 2016 ; Mehra and Kumar 2021 ; Singh and Ali 2021 ). The promises and opportunities for agricultural applications of genome editing are many, but farmer access depends on the regulatory processes in place. For example, many GnEd applications in animals being developed are for disease and insect control and introduction of these traits would reduce losses by farmers, improve animal health and welfare, and potentially reduce the need for antibiotics or insecticides. Other traits are focused on creating healthier, safer food products and improving animal welfare. Still others aim to produce animals that are more resilient to a changing climate or reduce the environmental footprint of animal agriculture. Some examples of GnEd applications in animals being developed for food and agriculture include:
Editing the insulin-like growth factor binding protein-2b gene in rainbow trout to characterize protein-level function of duplicate genes, leading to faster and more efficient growth (Cleveland et al. 2018 );
Enhanced genetic control of the new world screwworm (Scott 2014 , 2016 , 2019 , 2020 , 2021 ), a devastating pest for animals;
Livestock resistant to diseases such as mastitis (Donovan et al. 2014 ; Ramsay et al. 2017 ), bovine tuberculosis (Sonstegard 2018 ), and swine influenza (Kim 2019 ; Vincent 2019 );
Climate-adaptation traits such as heat tolerant cattle (Sonstegard 2016 );
Animal welfare-associated traits such as elimination of the need to castrate pigs (Donovan 2013 ; Maga et al. 2018 ; Berger et al. 2019 ) or to dehorn cattle (Sonstegard and Murray 2015 ; van Eenennaam 2017b );
Surrogate sires as a breeding tool for preserving germplasm and disseminating improved genetics (Ciccarelli et al. 2020 ), including applications in developing countries where use of conventional breeding tools such as artificial insemination have proven challenging (Patel 2021 ); and
Gender selection in cattle (van Eenennaam 2018 ) for improved production efficiency.
Investing in animal biotechnology research
Public investment in agricultural research provides economic benefits with annual rates of return between 20 and 60% (Fuglie and Heisey 2007 ). To advance animal biotechnology, investment is needed at all stages of development, including (1) basic research to discover new biotechnology tools; (2) method development to adapt tools to different species; (3) product development, including gene function identification and testing of traits in production settings; and (4) assistance with commercialization, including any required testing for regulatory approvals. Such investment is necessary in part to ensure that farmers in the United States can adapt to new challenges, but also to address global agricultural challenges and to ensure continued competitiveness in the global marketplace.
Many countries are increasing agricultural research investment (Clancy et al. 2016 ), including for GnEd in animals. However, in the United States, the percentage of federal research funding spent on agriculture declined from 40% in 1940 to just 2% in 2020 (Rowley 2020 ; AAAS 2021 ). Research related to agriculture is supported by many federal agencies (Jahn 2020 ); however, it is significant that only USDA has a mandate to research plants and livestock for the purposes of improving and protecting agriculture. The USDA Science Blueprint includes genome editing of plants and animals as a research priority for 2020 through 2025 (USDA 2019 ), but funding remains limited.
As described above, USDA agencies have a proven history of delivering quality animal breeding advances that have been useful for farmers. However, USDA projects involving biotechnology are distributed across many subject areas, so determining the number of USDA-ARS and NIFA projects or the level of funding is challenging. For example, in 2021, USDA-ARS budget levels for livestock production and livestock protection were $127 million and $124 million, respectively (USDA 2021 ), but existing databases lack the capability to list projects having to do with animal biotechnology or with GnEd specifically. Enhanced cataloging of biotechnology research will enable better classification and coordination of resources at USDA and across federal research funding agencies.
The 2018 Farm Bill established a 3-year pilot program called the Agriculture Advanced Research and Development Authority (AgARDA) for agricultural research and development. Funding for AgARDA was set at $50 million per year. The goals of AgARDA were to (1) prevent, prepare for, and protect against unintentional and intentional threats to U.S. agriculture and food; (2) enhance export competitiveness, environmental sustainability, and resilience to extreme weather; (3) enhance U.S. leadership in research that increases economic opportunities and security for farmers, ranchers, and rural communities; and (4) undertake research and development in areas that industry is not likely to pursue due to technological or financial uncertainty (U.S. House 2018a ). Extending AgARDA into a permanent program could complement existing research programs to help USDA advance animal biotechnology along with other agricultural research goals. In 2021, the Tri-Societies Footnote 2 expressed continued support for AgARDA and argued for expanding the pilot into an Advanced Research Projects Agency (ARPA) program to fund large, heavily-managed projects that would not otherwise be funded by USDA or the ARPA agencies at the Department of Defense, Department of Energy, or HHS (McMurray et al. 2021 ).
Such structural and organizational changes as well as additional investment could facilitate agricultural innovation, particularly for animal biotechnology, and help meet challenges such as global climate change and food security. For example, to improve product development and commercialization, there is a need to continue to fund and utilize existing programs for public private partnerships, such as the Foundation for Food & Agriculture Research (FFAR), which pairs federal funding with private funding to address complex agricultural challenges; the USDA Small Business Innovation Research (SBIR) program, which provides small, short-term grants to help bring innovations to commercialization; and the Office of Technology Transfer (OTT), which helps move USDA-ARS research discoveries to the market to solve agricultural problems and expand the economic impact of USDA-ARS research and development. There are also USDA research grants targeted at addressing agricultural challenges in developing countries, including a competitive grants program for research institutions in developing countries to develop agricultural biotechnology (U.S. House 2002 ). Many applications of USDA-supported GnEd research are well-suited to developing countries; some researchers are already partnering with foreign research institutions and not-for-profit organizations to deliver GnEd solutions to help alleviate poverty and address threats to animal agriculture, such as climate change (Ghosh 2019 ; Sonstegard 2016 ).
Public acceptance and market challenges to animal innovation
Significant public acceptance and market challenges must be addressed before GnEd in animals and the resulting products can be commercialized. These market challenges are driven in part by consumer concerns dating back to the introduction of transgenic crops in the 1990s and are influenced by GMO disinformation campaigns. Consumer purchasing decisions are complex; when asked to name concerns about food, consumers identify primary concerns such as taste, price, healthfulness, and convenience (Lusk et al. 2011 ), followed by secondary concerns such as animal welfare and sustainability concerns, including pesticides; biotechnology is only important to a small percentage of consumers (e.g., Armstrong et al. 2021). Consumers have a very low level of awareness of GnEd, and generally more positive attitudes about GnEd compared to GMOs (Beghin and Gustafson 2021 ), which may provide opportunities for education. While providing information about a technology can reinforce negative beliefs (Grunert 2002 ), consumers may be more willing to accept or even pay an increased price for products of GnEd when specific benefits are described (Tallapragada et al. 2021 ; Caputo et al. 2020 ).
When evaluating new biotech plants, regulatory systems generally compare food and environmental safety for plants developed using biotechnology with similar conventionally bred plants. While regulators do consider plant health, the safety of a genetic modification to the plant itself has not been a concern of the public. In contrast, the safety of a genetic modification to the animal is of greater interest to the public, as many people have strong emotional connections to animals and express concern for the welfare of farmed animals, although many have little understanding of modern animal production. Consumers may view GnEd or other biotechnologies as allowing for more intensive animal production and may need reassurance about the welfare of GnEd animals (DEFRA 2021 ). Conversely, public support for GnEd use in animals may increase if specific traits are demonstrated to have a positive impact on animal health or welfare, and thus be more acceptable to informed consumers (Tallapragada et al. 2021 ).
Commercialization decisions by developers and adoption decisions by producers depend on their ability to predict consumer attitudes towards GnEd and other animal biotechnologies. Food manufacturers and retailers may elect to not sell the products of animals or animal lineages in which GnEd was utilized, or to provide “non-GMO”-type labels for animal products produced without GnEd. Markets may choose to self-impose labeling and product segregation systems, even if not mandated, in response to perceived or real consumer demand. Transparency in the development, regulation, production, and commercialization of GnEd in animals—and a focus on GnEd traits that address societal needs—is essential in bringing innovations developed with GnEd to consumers.
Regulatory policy for animal biotechnology
The primary role of regulation is to protect public health and safety. Regulatory risk assessments consider scientific characteristics of a product or group of products, and may include similarity to conventional products; toxicological evaluation of a product or components of a product; investigation of potential environmental impacts; and exposure via food, feed, or in the environment (National Academies of Sciences, Engineering, and Medicine 2016 ). Regulatory approaches may be standardized for groups of products or determined on a case-by-case basis. Regulatory decisions may be informed by assessments conducted by other countries or groups of countries.
The United States is unusual in not having GMO laws. U.S. federal oversight for biotechnology is a coordinated framework in which each relevant federal regulatory agency implements its authority under existing laws (Unified Website for Biotechnology Regulation 2021 ), resulting in different processes for plants and animals. Most countries have specific GMO laws, with the same laws governing all organisms—plants, animals, and microorganisms. GMO regulations focus on the process used to create a product, rather than the characteristics of the product itself (Hallerman et al. 2022 ). Canada has the only purely product-based approach. In some countries, products of GnEd may face additional regulatory requirements beyond those required for similar products created via conventional breeding, even though the final food products may not be substantively different. This could result in products with similar characteristics being regulated differently within a single country.
In addition to regulatory risk assessments for products under GMO laws, some countries have an additional layer where a political-level decision is made for each product or group of products. Sometimes this is part of the official process, as in the European Union (EU), where the scientific risk assessment is separated from the approval decision (USDA-FAS 2020 ). Political involvement can also be ad hoc, such as when language directed at genetically engineered salmon Footnote 3 was included in U.S. Congressional spending bills (U.S. House 2016 , 2017 , 2018b , 2019 ). Political-level decisions may or may not be based on the regulatory assessment and might consider issues such as concerns of consumers, needs of domestic producers, and potential economic impacts both domestically and abroad (Smith et al. 2021 ).
For biotechnology regulations to be truly effective, they must also allow for safe products to be used by farmers and the public. Effective regulatory approaches are transparent, science-based, and risk-proportionate, as well as appropriate for intended use (e.g., an approach designed for biomedical products may not be appropriate for food and agricultural products, since the conditions of use are quite different). Effective regulatory approaches help instill public trust in our food supply and encourage innovation, if regulatory processes are defensible and credible to the public, whose views may reflect values-based issues that fall outside the realm of science-based regulation.
To encourage innovation, regulatory processes must be both timely and predictable. Clearly defined regulatory requirements can inform development and direct research approaches taken and the path to market. Similar phenotypes could be created via different GnEd approaches, and, under some regulatory schemes, different GnEd approaches used to create a specific phenotype could result in different regulatory requirements being applied. For example, under some regulatory approaches, a deletion of genetic material could result in the animal being regulated as a conventional animal, while use of a template (or some types of templates) to achieve a similar phenotype could require additional assessment under GMO regulations. Providing clear information to developers regarding regulatory requirements and exclusions for GnEd applications in animals before they are created can help streamline the development process and can reduce the cost of development, as well as minimize the number of animals required for development.
Regulatory approaches should account for specific characteristics of animal breeding that are not present in plant breeding. Inbreeding can have a negative effect on livestock and including GnEd as part of a breeding program to introduce new phenotypes could reduce inbreeding compared to conventional breeding programs (Mueller et al. 2019 , 2021 ). For example, regulatory paradigms that require lineage-level approval as opposed to species-level approval will lead to GnEd being cost prohibitive for many livestock and poultry species. Lineage-level approval will either preclude the use of GnEd or force breeders to sacrifice genetic diversity through inbreeding schemes necessary to utilize the few lineages put forward for approval. In swine and poultry breeding programs, organized as multi-layered pyramids, only a small group of founder animals needs to be GnEd. The flatter structures of cattle, sheep, and goat programs will require larger numbers of breeding animals to be edited. If regulatory systems do not allow for approved GnEd traits to be utilized across the target species, minor or rare breeds of economic or cultural value are particularly at risk of being excluded from access to GnEd opportunities. The regulatory approaches that countries choose will determine the availability of GnEd solutions to pressing animal health or environmental challenges.
There are additional impacts of regulatory approaches that may not be as apparent. In conventional breeding programs for food animals, products from animals in breeding pyramids can enter the food chain, allowing breeders to bring in revenue from the sale of animals as new traits are introduced and phenotypes are selected (Van Eenennaam et al. 2021 ). The sale of food products from conventional research animals at academic and government research institutions helps to support the high cost of animal research.
This has not been the case for most animals created via biotechnologies, including GnEd. The inability for these animals to enter the food chain under some regulatory approaches results in a significant loss of income. Not only are healthy livestock disposed of rather than used for food, but the developer must pay additional costs for the disposal of animals. This is particularly difficult for public sector researchers and represents a barrier to innovation. There is also an emotional cost for researchers and breeders when healthy animals must be destroyed. When non-risk-proportionate regulatory approaches are applied to genome edited animals that are healthy and would otherwise be deemed safe to eat during slaughter inspections, potentially inexpensive solutions to the agricultural challenges faced by the livestock, poultry, and aquaculture sectors will remain out of reach.
Conversely, regulation under existing, stringent food safety laws in place for conventional animals would help to defray the cost of development and reduce waste of wholesome animal products. U.S. regulators recognized that the initial regulations put in place for genetically engineered organisms would be burdensome, especially for academic institutions, developing countries, and small businesses (Maryanski 2006 ). Footnote 4 Modernization of regulatory approaches for these products is necessary, particularly to enable smaller companies and academic institutions to transfer the solutions they develop with GnEd into the hands of farmers and consumers.
Impacts of regulation on access
Countries’ regulatory approaches impact the technologies that are available to developers, the products that are developed, and the products that are available to farmers and consumers. The high costs and lengthy timeframe for regulatory approval, along with campaigns by some consumer groups organized against genetically engineered products, have steered breeding companies away from investment in transgenic approaches. Use of these technologies in animals has been largely limited to academic studies of gene function. While some genetically engineered animals have been commercialized, most have been for non-food, non-agricultural applications, and no animals have been approved for production in conventional livestock or aquaculture facilities in the United States. Many research models have been created (Whitelaw et al. 2016 ) and transgenic, fluorescent fish are popular as aquarium pets.
Thus far, only one genetically engineered animal developed for food and agricultural use has been commercialized. Footnote 5 In 1989, AquaBounty Technologies created the AquAdvantage salmon, containing a growth hormone construct from Chinook salmon that improved feed conversion and reduced production time from 3 years to 18 months. In 2010, HHS-FDA determined this fish was safe for human consumption, but it was not approved for food use and import until 2015. In 2021, the genetically engineered fish entered the market in Canada and the United States and was approved for sale in Brazil.
Despite the lack of approvals, many types of livestock and species of fish were created via genetic engineering (Murray and Maga 2016 ; Van Eenennaam et al. 2021 ). In a well-known case, Environment Canada approved a pig that has less polluting manure in 2009 (Environment Canada and Health Canada 2009 ). The pig, called Enviropig, was of sufficient interest to farmers that Canadian pork producers contributed to its development. However, without a sufficiently viable path to market, it became apparent that the cost of regulatory approval would be many-fold greater than the initial research investments and the developers lost financial support before they could receive food use approval from Health Canada or HHS-FDA (Nickel 2021 ).
While animal farmers have been unable to benefit from advances in agricultural biotechnology, crop farmers have had access to traits developed with biotechnology since 1998. The United States is a top producer of genetically engineered crops, with 177 million acres planted in 2019. Globally, 470 million acres of genetically engineered crops were planted in 2019 by about 17 million farmers in 29 countries (ISAAA 2019 ). Access has been limited to a few high-value species, Footnote 6 but genetically engineered crops have helped to improve the socio-economic conditions of farmers, with global economic gains from biotech crops from 1996 to 2018 estimated at US$224.9B (ISAAA 2019 ). For animal farmers to reap such benefits, there not only needs to be the introduction of new biotechnology tools, such as genome editing, but also a paradigm shift of regulatory approaches.
New regulatory approaches for genome editing
With the advent of genome editing, the regulatory landscape is changing as many countries modernize existing regulatory approaches. Regulatory protection goals remain the same for all foods, whether derived from biotechnology or conventional breeding, with the top priority being to protect the safety of humans, animals, and the environment. New regulatory approaches increasingly focus more on the characteristics (and potential risks) of products of new technologies, rather than on the method used to create them. There is also an increased understanding of the importance of encouraging innovation and of allowing safe products to be transferred to farmers, which will advance addressing significant global challenges and threats to agriculture and food production.
There are essentially two potential regulatory scenarios for products developed with GnEd: (1) to regulate products of GnEd under existing GMO regulations with no exclusions; or (2) to regulate products of GnEd under regulations for conventional animals and products if they do not contain transgenic DNA sequences. If all products of GnEd are regulated by a country as GMOs, then it is likely that GnEd would only be financially feasible for a few high-value crops and few, if any, GnEd applications in animals or specialty crops will be available. However, if GnEd applications in animals that could be achieved via conventional breeding are regulated like products of conventional breeding, then more products, including livestock, vegetables, and fruits, would likely be developed by public institutions and smaller companies. Then more countries could be involved in the development of new products, and more traits would be targeted for regional agricultural problems and local consumer tastes.
Regulatory agencies around the world are considering when to regulate a genome edited product under their existing GMO regulatory process (see Fig. 3 for a summary of potential options). Regulators generally agree that natural mutations and mutagenesis (shown at the top in green on Fig. 3 ) are regulated as conventional products. Regulators in most countries also agree that transgenic products (shown at the bottom in red in Fig. 3 ) are subject to regulation under GMO laws. Regulatory agencies are now considering the types of edits that can be made with genome editing, which can be grouped into four categories shown in yellow in Fig. 3 : (1) small changes, including substitutions and deletions; (2) short, cisgenic insertions; (3) long, cisgenic insertions; and (4) transgenic insertions. The question is where to draw the regulatory line. For many countries, such as Argentina and Brazil, the line has been drawn between cisgenic changes and transgenic changes (as shown by the yellow dashed line on Fig. 3 ), such that cisgenic products above the line are regulated as conventional products and transgenic products below the line are regulated under GMO laws.
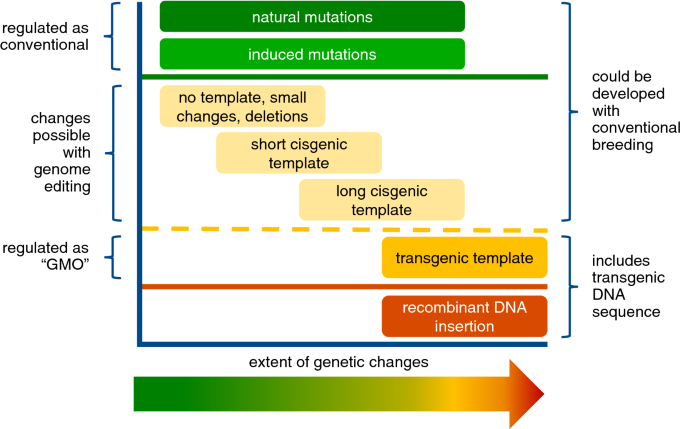
The type of genetic modifications and mutations that occur in organisms during conventional breeding (in green), in templated and untemplated genome editing (GnEd) applications, both cisgenic (in yellow) and transgenic (in orange), and in genetically engineered (GE) applications (in red)
While too few genetically engineered animals have been approved for agricultural use to draw conclusions about regulatory approaches, one can look to the evolution of regulatory approaches for biotech plants. In particular, new approaches taken by USDA-APHIS and the Ministry of Agriculture, Livestock and Fisheries in Argentina are informative.
Starting in 2011, USDA-APHIS offered the “Am I Regulated” (AIR) inquiry process to review the regulatory status of organisms developed with genetic engineering and determine if they met the definition of a “regulated article” under the legacy regulations (Hoffman 2021 ; USDA-APHIS 2020 ). In the petition process, developers could demonstrate that a regulated plant developed with genetic engineering did not pose a plant pest risk, and thus should not be regulated. There were clear differences between the types of developers submitting requests to each process, with less than 5% of AIR requests coming from major biotechnology companies and more than a third from public research institutions, including some from other countries (Fig. 4 ). There was also a clear difference in the types of plants and traits submitted. Most petition applications were for just two types of traits (herbicide tolerance and insect resistance) that were incorporated into a limited number of row crops. In contrast, the plant/trait combinations moving through the AIR process were much more diverse and included traits for disease resistance, improved flavor or nutrition, enhanced sustainability, and adaptations for climate change.
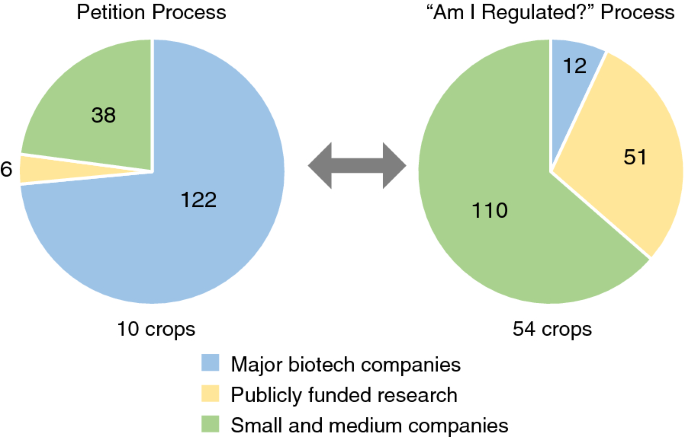
The types of developer utilizing the two USDA-APHIS legacy regulatory approaches: “Petition Process” (1992–2020) for genetically engineered on the left and “Am I Regulated? Inquiry Process” (2011–2020) on the right, which included 110 plants created via GnEd. Large biotechnology seed companies are shown in blue, small and medium biotechnology seed companies are shown in yellow, and publicly-funded research institutions developing plants with biotechnology are shown in green. USDA-APHIS adopted new processes in 2020
In 2020, USDA-APHIS adopted an updated regulatory approach for genetically engineered organisms that improved risk-proportionate regulation and phased out both AIR and petition processes (Hoffman 2021 ). Although the numbers are currently still small, the new Confirmation Request process appears to allow more small companies and academic institutions to enter the regulatory process, rather than only major biotechnology companies. Herbicide tolerance and insect resistance continue to be important traits to developers and farmers, but now these traits are just two of many that are moving forward through the new USDA-APHIS process, along with GnEd applications in crops developed with public funding (USDA-APHIS 2021 ).
In 2015, Argentina became the first country to publish their regulatory approach for genome editing, excluding cisgenic products from their GMO regulations (Whelan and Lema 2015 ). In 2018, Argentina also became the first country to determine that a certain genome edited animal was a conventional animal and therefore did not require additional GMO regulatory assessments. Argentina’s approach for genome edited animals is a relatively straightforward decision tree, in which products of genome editing with a new combination of genetic material (sometimes referred to “foreign DNA”) are regulated under their existing GMO regulations; otherwise, products are excluded and do not require additional regulation beyond those required for conventional animals. To further stimulate innovation, Argentina provides developers with a determination prior to development of new GnEd applications, so developers can know in advance of devoting resources to creating GnEd innovations whether the resulting animals and their products would be regulated under conventional or GMO regulations.
In 2020, Argentina published a socioeconomic study on their new regulatory approach that showed similar results as those observed by USDA-APHIS. Foreign multinational companies submitted 90% of products through the former regulatory process, but only 9% of products in the new process. Similar to USDA-APHIS results, 90% of products going through Argentina’s GMO approval process were a few high-value row crops with enhanced herbicide tolerance and insect resistance. Under the new approach, applications were more diverse, including traits focused on consumer preference and health benefits (Whelan et al. 2020 ). Argentina’s new regulatory approach facilitated applications from local companies, public researchers, and foreign small and medium companies, and more products were able to enter the regulatory process for the first time. The new policy brought new domestic and international innovation to Argentina.
Many countries with GMO laws have recently adopted approaches similar to Argentina’s approach. Brazil and Japan have made “non-GMO” determinations for fish with genomic deletions that increase muscle yield. In 2021, Japan became the first country to have food from a GnEd application in animals, a genome edited lineage of sea bream, marketed to the public (USDA-FAS 2021 ); a second GnEd application in tiger puffer fish also completed the notification process and is cleared for commercialization (MHLW 2021 ). The Japanese Ministry of Health, Labor, and Welfare and the Ministry of Agriculture, Forestry, and Fisheries deemed that these genome edited fish lineages have genetic changes that could have occurred via conventional breeding. These fish are the result of public funding and a partnership between universities and a small start-up company that formed in 2019 (Regional Fish Institute 2021 ). These countries are utilizing new regulatory approaches for GnEd applications in animals that could have been created via conventional breeding under the same food and environmental safety approaches used for conventional animals. This is allowing for GnEd applications in animals developed by public institutions to be commercialized quickly and holds promise for facilitating GnEd solutions developed with public funds to reach farmers and address agricultural challenges in the future.
Regulatory impacts on global trade
To enter any country’s market, a product must meet its regulatory requirements and ongoing market requirements. Differing regulatory policies for genome editing and asynchronous approvals for specific GnEd applications in animals across countries have the potential to disrupt global trade in animal products (Qaim 2020 ). Access to international markets is critical to the US animal agriculture. Exports of beef, pork, and broilers in 2021 accounted for 15.0%, 29.4%, and 16.4% of U.S. production, respectively (USDA-ERS 2022 ). Therefore, domestic adoption of GnEd applications in animals is contingent on continued access to foreign markets.
Lack of harmonization in market entry requirements such as labeling, product traceability, and segregation requirements will complicate trade and have the potential to stifle innovation. In order to maintain access to foreign markets, animal producers who utilize GnEd will need to conform to the most restrictive requirements of trading partners or develop costly segregation systems for their products. The livestock and poultry industries have historically been reluctant to segment products without significant financial incentives. Foreign markets that are outliers in terms of their regulatory approach for products of GnEd or other market requirements will either drive industry decisions globally if the market is of high value, or risk being cut off from global trade in animal products if the market is not of high value. Some countries have adopted or are considering regulatory policies that would allow for certain GnEd applications in animals and resultant animal products to enter domestic markets without segregation or additional labeling to distinguish them from other animals and their products.
Non-harmonized regulatory approaches in some countries may create challenges for domestic use of GnEd innovations. For example, in the EU all GnEd applications are currently subject to EU GMO regulations, and product segregation would be difficult, especially in cases where the products of GnEd cannot be reliably distinguished from other products by detection methods. While several EU Member States and members of the EU scientific community advocate for biotechnology regulatory reform within the EU (Turnbull et al. 2021 ) this effort may initially be limited to plants. The extent to which the EU remains a global outlier will impact adoption of GnEd, but there is hope: a European Commission study questioned whether existing GMO laws are “fit for purpose” when it comes to new breeding techniques like genome editing, and called for additional policy action, particularly for products that could have been developed with conventional breeding (European Commission 2021 ).
Hope for the future of animal biotechnology
There currently exists a great deal of hope that GnEd solutions will be delivered into the hands of farmers. We do not wish to discount this optimism but note a similar optimism regarding animal biotechnology in the 1980s, 1990s, and the early 2000s. At that time, researchers and the animal industry expected that the products of rDNA technologies would be allowed on the market. The animal industry was preparing for the expected entry of many new animals and products, but only one Footnote 7 ever made it to farmers. Both the plant and animal sectors have benefited greatly from advances in genomics, but only certain crops benefited from advances in genetic engineering. Hopefully, history will play out differently for genome editing.
Farmers and ranchers know what works best for their farms. If empowered to do so, many will choose genome editing, in concert with other technologies and conventional breeding, to enhance efforts in increasing productivity of farms and reducing environmental impacts. Responsible and supportive regulation of genome editing technologies will enable animal breeders to more rapidly and successfully develop enhanced breeds with desirable traits; reduce impacts of pests and pathogens; adapt to environmental impacts of climate change; reduce costs and numbers of animals required for genetic improvement; improve animal well-being; maintain product quality; and foster food safety (Rexroad et al. 2019 ). However, farmers and ranchers will not be able to access these tools unless flexible approaches are in place that encourage innovation, allow their wide use within animal populations, and allow safe products to be used on farms. Regulatory approaches and how they are applied have a tremendous impact in determining what products are developed and who can afford to use new technologies. The livelihoods of people all along the agricultural value chain depend upon the policy choices made by their own country and by other countries.
We hope all countries can agree that regulatory approaches should allow farmers to access GnEd solutions developed at public research institutions, and that any regulatory requirements should facilitate the 3Rs of animal research (replace, reduce, refine) in a way that is efficient. This will ensure that GnEd solutions are available in time to address the problems for which they were created, such as climate change, disease and pest threats, improving animal welfare, or increasing food safety and security. The global regulatory landscape for products of genome editing is rapidly evolving, with an increasing number of countries focusing more on characteristics of products and whether they could be achieved by conventional breeding, rather than the technologies used to create them. Some countries are already moving forward, allowing the marketing of food products from GnEd applications in animals developed with public support. Other countries should follow their lead in developing approaches that treat low risk applications as low risk, allowing farmers around the world to compete fairly.
We find ourselves at a crossroads in the United States today. Many in the U.S. animal agriculture sector have argued that without major policy changes, no animal biotechnology solutions are likely to be available to U.S. farmers and ranchers, putting them at a disadvantage to farmers in other countries. Historically, the United States has been a global leader in development of improved agricultural breeds and breeding tools to enhance genetic gains in fish and livestock. Enabling U.S. researchers and breeders to be leaders in incorporating GnEd tools into our breeding programs will require research investment and appropriate market transparency, and effective regulatory policies are essential to enable safe products to reach the market. Regulatory policies should encourage innovation and provide the opportunity to integrate new innovations into current agricultural production systems. We need to step forward and look to the future so that the next generation of farmers and breeders has more options, not fewer, to better meet current and future challenges, and to do so more sustainably.
Availability of data and materials
Not applicable.
These definitions may differ from colloquial use of the terms, and from how countries define these terms under their biotechnology regulations. Gene editing is often colloquially used interchangeably with the term genome editing.
American Society of Agronomy (ASA), Crop Science Society of America (CSSA), and Soil Science Society of America (SSSA).
Language in Consolidated Appropriation Acts of 2016–2019 under Division—Agriculture, Rural Development, Food and Drug Administration, and Related Agencies Appropriations Acts; Title VII, General Provisions: “During fiscal year 201x, the Food and Drug Administration shall not allow the introduction or delivery for introduction into interstate commerce of any food that contains genetically engineered salmon until the FDA publishes final labeling guidelines for informing consumers of such content.”.
2006 interview of the HHS-FDA Biotechnology Coordinator stating that “The foods developed by this technology [GE] undergo far more testing than all the other foods that enter the grocery store, for food safety. There’s really a huge burden that’s placed on the developers to use this technology, and that is going to be an issue for developing countries and an issue for small companies. It is, in fact, scientifically difficult to justify a lot of the testing that is being done today for these foods in terms of the public health issues that they actually don’t raise. But most of this is now being done to provide confidence to the public that the foods are safe.”.
In 2020, HHS-FDA approved a second animal for food use, the GalSafe pig; but this animal was developed for biomedical purposes, such as kidney xenotransplants.
The ISAAA GM Approval Database lists 32 crop species approved for food, feed, and environmental release, but only 14 were planted in 2019. Four major biotech crops were planted on 99% of the total biotech crop area in 2019: soybeans, maize, cotton, and canola (ISAAA 2019 ).
HHS-FDA approved recombinant bovine somatotropin (rbST) for use as an animal drug in 1993.
Abbreviations
Agriculture and Food Research Initiative
Agriculture Advanced Research and Development Authority
Am I Regulated
Animal and Plant Health Inspection Service
Advanced Research Projects-style research agency
Agricultural Research Service
United Kingdom Department for Environment, Food and Rural Affairs
Economic Research Service
European Union
Foreign Agricultural Service
Food and Drug Administration
Foundation for Food & Agriculture Research
Genetic engineering
Genetically modified
Genetically modified organism
Genome editing
U.S. Department of Health and Human Services
International Service for the Acquisition of Agri-biotech Applications
Japanese Ministry of Health, Labor, and Welfare
National Institute of Food and Agriculture
Office of Technology Transfer
Recombinant DNA
Small Business Innovation Research
U.S. Department of Agriculture
AAAS. Federal R&D budget dashboard. https://www.aaas.org/programs/r-d-budget-and-policy/federal-rd-budget-dashboard . Accessed 24 Nov 2021.
Armstrong B, King L, Clifford R, Jitlal M. Food and You 2: Wave 1 Key Findings. Food Standard Agency. United Kingdom. 2021. https://www.food.gov.uk/sites/default/files/media/document/fy2-wave-1-report-_key-findings_1.pdf . Accessed 16 Feb 2022.
Babb D. History of the Mule. American Mule Museum. 2021. https://www.mulemuseum.org/history-of-the-mule.html . Accessed 23 Nov 2021.
Beghin JC, Gustafson CR. Consumer valuation of and attitudes towards novel foods produced with new plant engineering techniques: a review. Sustainability. 2021;13:11348. https://doi.org/10.3390/su132011348 .
Article CAS Google Scholar
Berger TJ, Maga EA, Ross PJ, Denicol AN, Horback, KR, Yang, XI. Boar meat without boar taint: a model. Accession no: 1018166. USDA National Institute of Food and Agriculture, Washington, DC. 2019. https://cris.nifa.usda.gov/cgi-bin/starfinder/0?path=fastlink1.txt&id=anon&pass=&search=(AN=1018166)&format=WEBLINK . Accessed 6 Dec 2021.
Bleck GT, White BR, Miller DJ, Wheeler MB. Production of bovine alpha-lactalbumin in the milk of transgenic pigs. J Anim Sci. 1998;76:3072–8. https://doi.org/10.2527/1998.76123072x .
Article CAS PubMed Google Scholar
Campbell KHS, McWhir J, Ritchie WA, Wilmut I. Sheep cloned by nuclear transfer from a cultured cell line. Nature. 1996;380:64–6. https://doi.org/10.1038/380064a0 .
Cao W, Mays JK, Kulkarni G, Dunn JR, Fulton RM, Fadly AM. Further observations on serotype 2 Marek’s disease virus-induced enhancement of spontaneous avian leukosis virus-like bursal lymphomas in ALVA6 transgenic chickens. Avian Pathol. 2015;44:23. https://doi.org/10.1080/03079457.2014.989195 .
Article PubMed Google Scholar
Caputo V, Lusk J, Kilders V. Consumer Acceptance of Gene Edited Foods: a nationwide survey on US consumer beliefs, knowledge, understanding, and willingness to pay for gene-edited foods under different treatments. 2020. FMI Foundation report. https://foodinsight.org/2021-food-health-survey/ . Accessed 5 Dec 2021.
Ciccarelli M, Giassetti MI, Miao D, Oatley MJ, Robbins C, Lopez- Biladeau B, Waqas MS, Tibary A, Whitelaw B, Lillico S, Park CH, Park KE, Telugu B, Fan Z, Liu Y, Regouski M, Polejaeva IA, Oatley JM. Donor-derived spermatogenesis following stem cell transplantation in sterile NANOS2 knockout males. Proc Natl Acad Sci USA. 2020;117:24195–204. https://doi.org/10.1073/pnas.2010102117 .
Article CAS PubMed PubMed Central Google Scholar
Clancy M, Fuglie K, Heisey P. U.S. Agricultural R&D in an Era of Falling Public Funding. Amber Waves. 2016. USDA, Economic Research Service. https://www.ers.usda.gov/amber-waves/2016/november/us-agricultural-r-d-in-an-era-of-falling-public-funding/ . Accessed 5 Dec 2021.
Cleveland BM, Yamaguchi G, Radler LM, Shimizu M. Editing the duplicated insulin-like growth factor binding protein-2b gene in rainbow trout ( Oncorhynchus mykiss ). Sci Rep. 2018;8:16054. https://doi.org/10.1038/s41598-018-34326-6 .
DEFRA (United Kingdom Department for Environment, Food and Rural Affairs). Summary of responses to a consultation on the regulation of genetic technologies. 2021. https://assets.publishing.service.gov.uk/government/uploads/system/uploads/attachment_data/file/1021309/genetic-technologies-regulation-summary-of-responses.pdf . Accessed 5 Dec 2021.
Donovan DM. Genetically modified livestock via zygotic genome editing. Accession no: 0425211. USDA National Institute of Food and Agriculture, Washington, DC. 2013. https://cris.nifa.usda.gov/cgi-bin/starfinder/0?path=fastlink1.txt&id=anon&pass=&search=(AN=0425211)&format=WEBLINK . Accessed 6 Dec 2021.
Donovan DM, Park C, Swift S, Telugu B. Creation of novel CRISPR antimicrobials and genomic integration of peptidoglycan hydrolase antimicrobials via genome editing with CRISPR technology. Accession no: 0426737. USDA National Institute of Food and Agriculture, Washington, DC. 2014. https://cris.nifa.usda.gov/cgi-bin/starfinder/0?path=fastlink1.txt&id=anon&pass=&search=(AN=0426737)&format=WEBLINK . Accessed 6 Dec 2021.
Dunham RA, Warr GW, Nichols A, Duncan PL, Argue B, Middleton D, Kucuktas H. Enhanced bacterial disease resistance of transgenic channel catfish Ictalurus punctatus possessing cecropin genes. Mar Biotechnol (NY). 2002;4:338–44. https://doi.org/10.1007/s10126-002-0024-y .
Environment Canada and Health Canada. Risk assessment summary conducted pursuant to the new substances notification regulations (Organisms) of the Canadian Environmental Protection Act, 1999. NSN 15676: Cassie line of genetically modified Sus scrofa Domestica. Nov 26, 2009. https://www.canada.ca/content/dam/eccc/migration/main/subsnouvelles-newsubs/5b919791-d9a0-48ec-a4a1-7b2fa8937d6e/nsn-2015676-20-20en.pdf . Accessed 23 Nov 2021.
European Commission. Study on the status of new genomic techniques under Union law and in light of the Court of Justice ruling in Case C-528/16. SWD(2021) 92 final. Brussels: European Commission. 2021. https://ec.europa.eu/food/plants/genetically-modified-organisms/new-techniques-biotechnology/ec-study-new-genomic-techniques_en . Accessed 6 Dec 2021.
Fuglie KO, Heisey PW. Economic returns to public agricultural research. EB-10. USDA Economic Research Service. 2007. https://www.ers.usda.gov/webdocs/publications/42826/11496_eb10_1_.pdf?v=1238.7 . Accessed 5 Dec 2021.
Garcia ALS, Bosworth B, Waldbieser G, Misztal I, Tsuruta S, Lourenco DAL. Development of genomic predictions for harvest and carcass weight in channel catfish. Genet Sel Evol. 2018;50:66. https://doi.org/10.1186/s12711-018-0435-5 .
Article PubMed PubMed Central Google Scholar
Ghosh P. Gene-edited animal plan to relieve poverty in Africa. BBC News. 2019. https://www.bbc.com/news/science-environment-47197896 . Accessed 7 Dec 2021.
Go Brangus. History of the International Brangus Breeding Association. 2008. https://gobrangus.com/wp-content/uploads/2014/08/02HISTORY_OF_IBBA.pdf . Accessed 23 Nov 2021.
Golding MC, Long CR, Carmell MA, Hannon GJ, Westhusin ME. Suppression of prion protein in livestock by RNA interference. PNAS. 2006;103:5285–90. https://doi.org/10.1073/pnas.0600813103 .
Green RD, Qureshi MA, Long JA, Burfening PJ, Hamernik DL. Identifying the future needs for long-term USDA Efforts in agricultural animal genomics. Int J Biol Sci. 2007;3:185–91. https://doi.org/10.7150/ijbs.3.185 .
Grunert KG. Current issues in the understanding of consumer food choice. Trends Food Sci Technol. 2002;13:275–85.
Hammer RE, Pursel VG, Rexroad CE, Wall RJ, Bolt DJ, Ebert KM, Palmiter RD, Brinster RL. Production of transgenic rabbits, sheep and pigs by microinjection. Nature. 1985;315:680–3. https://doi.org/10.1038/315680a0 .
Hallerman EM, Bredlau JP, Camargo LSA, Dagli MLZ, Karembu M, Ngure G, Romero-Aldemita R, Rocha-Salavarrieta PJ, Tizard M, Walton M, Wray-Cahen D. Towards progressive regulatory approaches for agricultural applications of animal biotechnology. Transgenic Res. 2022. https://doi.org/10.1007/s11248-021-00294-3 .
Hoffman NE. Revisions to USDA biotechnology regulations: the SECURE rule. Proc Nat Acad Sci. 2021;118:e2004841118. https://doi.org/10.1073/pnas.2004841118 .
Hunton P. 100 years of poultry genetics. World’s Poultry Sci J. 2006;62:417–28. https://doi.org/10.1079/WPS2006104 .
Article Google Scholar
ISAAA (International Service for the Acquisition of Agri-biotech Applications). Global status of commercialized biotech/GM Crops in 2019: biotech crops drive socio-economic development and sustainable environment in the new frontier. ISAAA Brief No. 55. ISAAA: Ithaca, NY; 2019.
Jahn M. New solutions for a changing climate: The policy imperative for public investment in agriculture R&D. Chicago Council on Global Affairs. 2020. https://www.thechicagocouncil.org/sites/default/files/2020-12/report_new-solutions-for-changing-climate_0.pdf . Accessed 24 Nov 2021.
Karavolias NG, Horner W, Abugu MN, Evanega SN. Application of gene editing for climate change in agriculture. Front Sustain Food Syst. 2021;5:685801. https://doi.org/10.3389/fsufs.2021.685801 .
Kasinathan P, Wei H, Xiang T, Molina JA, Metzger J, Broek D, Kasinathan S, Faber DC, Allan MF. Acceleration of genetic gain in cattle by reduction of generation interval. Sci Rep. 2015;5:8674. https://doi.org/10.1038/srep08674 .
Kim Y. Investigation of genetic factors for swine influenza a virus and porcine reproductive and respiratory syndrome virus. Accession no: 1019022. USDA National Institute of Food and Agriculture, Washington, DC. 2019. https://cris.nifa.usda.gov/cgi-bin/starfinder/0?path=fastlink1.txt&id=anon&pass=&search=(AN=1019022)&format=WEBLINK . Accessed 6 Dec 2021.
Livestock Conservancy: Beltsville Small White Turkey. 2021. https://livestockconservancy.org/heritage-breeds/heritage-breeds-list/beltsville-small-white-turkey/ . Accessed 23 Nov 2021.
Lusk JL, Roosen J, Shogren J. The Oxford handbook of the economics of food consumption and policy. Oxford: Oxford University Press; 2011.
Book Google Scholar
Maga EA, Shoemaker CF, Rowe JD, BonDurant RH, Anderson GB, Murray JD. Production and processing of milk from transgenic goats expressing human lysozyme in the mammary gland. J Dairy Sci. 2006;89:518–24. https://doi.org/10.3168/jds.S0022-0302(06)72114-2 .
Maga EA, Berger TJ, Horback, KM. Reduction of androgens by gene editing for the genetic containment of livestock. Accession no: 1017031. USDA National Institute of Food and Agriculture, Washington, DC. 2018. https://cris.nifa.usda.gov/cgi-bin/starfinder/0?path=fastlink1.txt&id=anon&pass=&search=(AN=1017031)&format=WEBLINK . Accessed 6 Dec 2021.
Maryanski J. History of the U.S. Food and Drug Administration. Oral history interview with James Maryanski. 2006. https://www.fda.gov/media/83918/download . Accessed 23 Oct 2021.
McMurray G, Murray S, Butler E, Northrup D, Odom L Stulberg E, Wendroth O. A roadmap for AgARDA at USDA. American Society of Agronomy. 2021. https://www.agronomy.org/files/science-policy/letters/2021-04-agarda-roadmap.pdf . Accessed on 7 Dec 2021.
Mehra VK, Kumar S. The application of CRISPR/Cas9 technology for farm animals: a review. Agric Rev. 2021. https://doi.org/10.18805/ag.R-2163 .
MHLW. Japanese Ministry of Health, Labor, and Welfare. List of foods and additives notified based on food hygiene guidelines for foods and additives applied to genome editing technology. 2021. https://www.mhlw.go.jp/stf/seisakunitsuite/bunya/kenkou_iryou/shokuhin/bio/genomed/newpage_00010.html . Accessed 23 Nov 2021. (In Japanese) .
Moser DW, Miller SP, Retallick KJ, Lu D, Kuehn LA. Genomic selection in the beef industry: current achievements and future directions. J Anim Sci. 2019;97(Suppl. 3; Abstr. 52):54–5. https://doi.org/10.1093/jas/skz258.110 .
Mueller ML, Cole JB, Sonstegard TS, Van Eenennaam AL. Comparison of gene editing versus conventional breeding to introgress the POLLED allele into the US dairy cattle population. J Dairy Sci. 2019;102:4215–26. https://doi.org/10.3168/jds.2018-15892 .
Mueller ML, Cole JB, Connors NK, Johnston DJ, Randhawa IAS, Van Eenennaam AL. Comparison of gene editing versus conventional breeding to introgress the POLLED allele into the tropically adapted Australian Beef Cattle Population. Front Genet. 2021;12:593154. https://doi.org/10.3389/fgene.2021.593154 .
Murray JD, Maga EA. Genetically engineered livestock for agriculture: a generation after the first transgenic animal research conference. Transgenic Res. 2016;25:321–7. https://doi.org/10.1007/s11248-016-9927-7 .
National Academies of Sciences, Engineering, and Medicine. Regulation of current and future genetically engineered crops. In: Genetically engineered crops: experiences and prospects. Washington: The National Academies Press; 2016. p. 455–522. https://doi.org/10.17226/23395 .
Nickel R. Death knell may sound for Canada's GMO pigs. Reuters. 2012. https://www.reuters.com/article/canada-us-gmo-canada-pigs-idCABRE83110320120402 . Accessed 19 Nov 2021.
Patel E. Scientists in Africa explore use of surrogate sires to improve small ruminant breeds. ILRI News. 2021. https://www.ilri.org/news/scientists-africa-explore-use-%E2%80%98surrogate-sires%E2%80%99-improve-small-ruminant-breeds . Accessed 24 Nov 2021.
Pryce J, de Haas Y. Genetic selection for dairy cow welfare and resilience to climate change. In: Webster J, editor. Achieving sustainable production of milk: volume 3: Dairy herd management and welfare. Cambridge: Burleigh Dodds Science Publishing Ltd; 2017. p. 81–102. https://doi.org/10.19103/as.2016.0006.04 .
Chapter Google Scholar
Pursel V, Rexroad C. Recent progress in the transgenic modification of swine and sheep. Mol Reprod Dev. 1993;36:251.
Pursel VG, Mitchell AD, Bee G, Elsasser TH, McMurtry JP, Wall RJ, Coleman ME, Schwartz RJ. Growth and tissue accretion rates of swine expressing an insulin-like growth factor I transgene. Anim Biotechnol. 2004;15:33–45. https://doi.org/10.1081/ABIO-120029812 .
Qaim M. Role of new plant breeding technologies for food security and sustainable agricultural development. Appl Econ Perspect Policy. 2020;42:129–50. https://doi.org/10.1002/aepp.13044 .
Ramsay TG, Powell A, Park C, Park K, Telugu B. CRISPR/cas mediated gene-targeting to reduce milk allergens and mastitis in goats. Accession no: 0432327. USDA National Institute of Food and Agriculture, Washington, DC. 2017. https://cris.nifa.usda.gov/cgi-bin/starfinder/0?path=fastlink1.txt&id=anon&pass=&search=(AN=0432327)&format=WEBLINK . Accessed 6 Dec 2021.
Regional Fish Institute. 2021. https://regional.fish/en/ . Accessed 6 Dec 2021.
Rexroad C, Vallet J, Matukumalli LK, Reecy J, Bickhart D, Blackburn H, Boggess M, Cheng H, Clutter A, Cockett N, Ernst C, Fulton JE, Liu J, Lunney J, Neibergs H, Purcell C, Smith TPL, Sonstegard T, Taylor J, Telugu B, Van Eenennaam A, Van Tassell CP, Wells K. Genome to phenome: improving animal health, production, and well-being—a new USDA Blueprint for Animal Genome Research 2018–2027. Front Genet. 2019. https://doi.org/10.3389/fgene.2019.00327 .
Richt J, Kasinathan P, Hamir AN, Castilla J, Sathiyaseelan T, Vargas F, Sathiyaseelan J, Wu H, Matsushita H, Koster J, Kato S, Ishida I, Soto C, Robl JM, Kuroiwa Y. Production of cattle lacking prion protein. Nat Biotechnol. 2007;25:132–8. https://doi.org/10.1038/nbt1271 .
Rowley LG. Public Agriculture Research: the United States can’t catch up by slowing down. 2020. The Lugar Center. https://www.thelugarcenter.org/newsroom-news-409.html . Accessed 6 Dec 2021.
Scott M. Development and evaluation of transgenic insect strains for genetic control programs. Accession no: 1005012. USDA National Institute of Food and Agriculture, Washington, DC. 2014. https://cris.nifa.usda.gov/cgi-bin/starfinder/0?path=fastlink1.txt&id=anon&pass=&search=(AN=1005012)&format=WEBLINK . Accessed 6 Dec 2021.
Scott M. Development and evaluation of safeguards for conditional suppressive gene drives for spotted wing drosophila and the new world screwworm. Accession no: 1010437. USDA National Institute of Food and Agriculture, Washington, DC. 2016. https://cris.nifa.usda.gov/cgi-bin/starfinder/0?path=fastlink1.txt&id=anon&pass=&search=(AN=1010437)&format=WEBLINK . Accessed 6 Dec 2021.
Scott M. Development of novel systems for enhanced genetic control of the new world screwworm. Accession no: 1020092. USDA National Institute of Food and Agriculture, Washington, DC. 2019. https://cris.nifa.usda.gov/cgi-bin/starfinder/0?path=fastlink1.txt&id=anon&pass=&search=(AN=1020092)&format=WEBLINK . Accessed 6 Dec 2021.
Scott M. Development and evaluation of y-linked gene editors for suppression of populations of spotted wing drosophila and the new world screwworm. Accession no: 1023744. USDA National Institute of Food and Agriculture, Washington, DC. 2020. https://cris.nifa.usda.gov/cgi-bin/starfinder/0?path=fastlink1.txt&id=anon&pass=&search=(AN=1023744)&format=WEBLINK . Accessed 6 Dec 2021.
Scott M. Assessing the influence of genetic background on the efficacy of drosophila suzukii male-only and gene drive strains. Accession no: 1027009. USDA National Institute of Food and Agriculture, Washington, DC. 2021. https://cris.nifa.usda.gov/cgi-bin/starfinder/0?path=fastlink1.txt&id=anon&pass=&search=(AN=1027009)&format=WEBLINK . Accessed 6 Dec 2021.
Siegel PB, Barger K, Siewerdt F. Limb health in broiler breeding: history using genetics to improve welfare. J Appl Poult Res. 2019;28:785–90. https://doi.org/10.3382/japr/pfz052 .
Singh P, Ali SA. Impact of CRISPR-Cas9-based genome engineering in farm animals. Vet Sci. 2021;8:122. https://doi.org/10.3390/vetsci8070122 .
Smith V, Wesseler JHH, Zilberman D. New plant breeding technologies: an assessment of the political economy of the regulatory environment and implications for sustainability. Sustainability. 2021;13:3687.
Sonstegard T. Development of tools for accelerating genetic improvement of livestock. (Accession no. 1008017). USDA National Institute of Food and Agriculture, Washington, DC. 2016. https://cris.nifa.usda.gov/cgi-bin/starfinder/0?path=fastlink1.txt&id=anon&pass=&search=(AN=1008017)&format=WEBLINK . Accessed 23 Nov 2021.
Sonstegard T. Tri-partite collaborative: targeted genome editing to understand and enhance genetic resistance to bovine tuberculosis in domestic cattle populations (target-tb). Accession no: 1015508. USDA National Institute of Food and Agriculture, Washington, DC. 2018. https://cris.nifa.usda.gov/cgi-bin/starfinder/0?path=fastlink1.txt&id=anon&pass=&search=(AN=1015508)&format=WEBLINK . Accessed 6 Dec 2021.
Sonstegard T, Murray JD. Improvement of diary animal well-being by genetic dehorning. Accession no: 1005738. USDA National Institute of Food and Agriculture, Washington, DC. 2015. https://cris.nifa.usda.gov/cgi-bin/starfinder/0?path=fastlink1.txt&id=anon&pass=&search=(AN=1005738)&format=WEBLINK . Accessed 6 Dec 2021.
Tallapragada M, Hardy BW, Lybrand E, Hallman WK. Impact of abstract versus concrete conceptualization of genetic modification (GM) technology on public perceptions. Risk Anal. 2021;41:976–91. https://doi.org/10.1111/risa.13591 .
Tan W, Proudfoot C, Lillico SG. Whitelaw CBA, Gene targeting, genome editing: from Dolly to editors. Transgenic Res. 2016;25:273–87. https://doi.org/10.1007/s11248-016-9932-x .
Taylor JB. Prelude to the Tremendous Targhee: the history behind the history. In: Targhee Talk: the Newsletter of the U.S. Targhee Sheep Association. p. 9–11. 2018. http://www.ustargheesheep.org/wp-content/uploads/2018/10/targheetalk55_oct2018.pdf . Accessed 23 Nov 2021.
Turnbull C, Lillemo M, Hvoslef-Eide TAK. Global regulation of genetically modified crops amid the gene edited crop boom—a review. Front Plant Sci. 2021;12:258.
Unified Website for Biotechnology Regulation. About the coordinated framework. 2021. https://usbiotechnologyregulation.mrp.usda.gov/biotechnologygov/about . Accessed 6 Dec 2021.
Upperman LR, Kinghorn BP, MacNeil MD, Eenennaam AL. Management of lethal recessive alleles in beef cattle through the use of mate selection. Genet Sel Evol. 2019;51:36. https://doi.org/10.1186/s12711-019-0477-3 .
USDA. USDA science blueprint: a roadmap for USDA Science from 2020 to 2025. 2019. https://www.usda.gov/sites/default/files/documents/usda-science-blueprint.pdf . Accessed 6 Dec 2021.
USDA. FY2021 budget summary. 2021. https://www.usda.gov/sites/default/files/documents/usda-fy2021-budget-summary.pdf . Accessed 6 Dec 2021.
USDA-APHIS: “Am I Regulated” Process. 2020. https://www.aphis.usda.gov/aphis/ourfocus/biotechnology/am-i-regulated/am_i_regulated . Accessed 16 Nov 2021.
USDA-APHIS. Animal and Plant Health Inspection Service. Confirmation Request Process. 2021. https://www.aphis.usda.gov/aphis/ourfocus/biotechnology/permits-notifications-petitions/confirmations . Accessed 16 Nov 2021.
USDA-ARS. ARS strategies to benefit aquaculture industry. AgResearch Magazine. 2018;66(1). https://agresearchmag.ars.usda.gov/2018/jan/industry/ . Accessed 26 Jan 2022.
USDA-ARS. Research Project # 8030-31000-005-000-D: Genetic Improvement of North American Atlantic Salmon and the Eastern Oyster for Aquaculture Production. 2019. https://www.ars.usda.gov/research/project/?accnNo=437429 . Accessed 26 Jan 2022.
USDA-ARS. Agricultural Research Service—about ARS. 2021. https://www.ars.usda.gov/about-ars . Accessed 16 Nov 2021.
USDA-ERS. Livestock, dairy, and poultry outlook: February 2022. LDP-M-332. USDA, Economic Research Service. https://www.ers.usda.gov/webdocs/outlooks/103284/ldp-m-332.pdf?v=12.4 . Accessed 16 Feb 2022.
USDA-FAS. Global Agriculture Information Network (GAIN) database. European Union: agricultural biotechnology annual. GAIN Report Number: E42020-0101. 31 Dec 2020. https://www.fas.usda.gov/data/european-union-agricultural-biotechnology-annual-0 . Accessed 5 Dec 2021.
USDA-FAS. Global Agricultural Information Network (GAIN) database. MHLW Publishes Considerations for Genome Edited Fish. GAIN Report Number: JA2021-0132. 28 Sept 2021. https://apps.fas.usda.gov/newgainapi/api/Report/DownloadReportByFileName?fileName=MHLW%20Publishes%20Considerations%20for%20Genome%20Edited%20Fish_Tokyo_Japan_09-22-2021 . Accessed 16 Nov 2021.
USDA-NIFA: Agriculture and Food Research Initiative (AFRI). 2021a. https://nifa.usda.gov/program/agriculture-and-food-research-initiative-afri . Accessed 05 Dec 2021.
USDA-NIFA. National Institute of Food and Agriculture—who we are. 2021b. https://www.nifa.usda.gov/who-we-are . Accessed 16 Nov 2021.
U.S. House. 99th Congress: H.R. 2100: Food Security Act of 1985. Washington: US Government Publishing Office. 1985. https://www.govinfo.gov/content/pkg/STATUTE-99/pdf/STATUTE-99-Pg1354.pdf . Accessed 5 Dec 2021.
U.S. House. 105th Congress: S. 1150 (H.R. 2534): Research, Extension, and Education Reform Act of 1998. Sec. 401. Washington: US Government Publishing Office. 1998. https://www.govinfo.gov/content/pkg/PLAW-105publ185/pdf/PLAW-105publ185.pdf . Accessed 5 Dec 2021.
U.S. House. 107th Congress: H.R. 2646: Farm Security and Rural Investment Act of 2002. Sec. 401. Washington: US Government Publishing Office. 2002. https://www.govinfo.gov/content/pkg/PLAW-107publ171/pdf/PLAW-107publ171.pdf . Accessed 5 Dec 2021.
U.S. House. 110th Congress: H.R. 2419: Food, Conservation, and Energy Act of 2008. Washington: US Government Publishing Office. 2008. https://www.govinfo.gov/content/pkg/PLAW-110publ234/pdf/PLAW-110publ234.pdf . Accessed 5 Dec 2021.
U.S. House. 113th Congress: H.R. 2642: Agricultural Act of 2014. Washington: US Government Publishing Office. 2014. Sec. 1433. https://www.govinfo.gov/content/pkg/PLAW-113publ79/html/PLAW-113publ79.htm . Accessed 5 Dec 2021.
U.S. House. 114th Congress: H.R. 2029: Consolidated Appropriations Act, 2016. Sec. 761. Washington: US Government Publishing Office. 2015. https://www.govinfo.gov/content/pkg/PLAW-114publ113/pdf/PLAW-114publ113.pdf . Accessed 5 Dec 2021.
U.S. House. 115th Congress: H.R. 244: Consolidated Appropriations Act, 2017. Sec. 761. Washington: US Government Publishing Office. 2017. https://www.govinfo.gov/content/pkg/PLAW-114publ113/pdf/PLAW-114publ113.pdf . Accessed 5 Dec 2021.
U.S. House. 115th Congress: H.R. 2: Agriculture Improvement Act of 2018a. Sec. 7132; amended Sec. 1473H. Washington: US Government Publishing Office. 2018a. https://www.govinfo.gov/content/pkg/PLAW-115publ141/pdf/PLAW-115publ141.pdf . Accessed 5 Dec 2021.
U.S. House. 115th Congress: H.R. 1625: Consolidated Appropriations Act, 2018b. Sec. 770. Washington: US Government Publishing Office. 2018b. https://www.govinfo.gov/content/pkg/PLAW-115publ141/pdf/PLAW-115publ141.pdf . Accessed 5 Dec 2021.
U.S. House. 116th Congress: H.J. Res. 31: Consolidated Appropriations Act, 2019. Sec. 776. Washington: US Government Publishing Office. 2019. https://www.govinfo.gov/content/pkg/PLAW-116publ6/pdf/PLAW-116publ6.pdf Accessed 5 Dec 2021.
Vallejo RL, Leeds TD, Gao G, Parsons JE, Martin KE, Evenhuis JP, Fragomeni BO, Wiens GD, Palti Y. Genomic selection models double the accuracy of predicted breeding values for bacterial cold water disease resistance compared to a traditional pedigree-based model in rainbow trout aquaculture. Genet Sel Evol. 2017;49:17. https://doi.org/10.1186/s12711-017-0293-6 .
Van Eenennaam AL. Genetic modification of food animals. Curr Opin Biotechnol. 2017a;44:27–34. https://doi.org/10.1016/j.copbio.2016.10.007 .
Van Eenennaam AL. Comparative evaluation of the phenotype, genome and animal products derived from offspring of a genome edited, hornless bull and controls. Accession no: 1013722. USDA National Institute of Food and Agriculture, Washington, DC. 2017b. https://cris.nifa.usda.gov/cgi-bin/starfinder/0?path=fastlink1.txt&id=anon&pass=&search=(AN=1013722)&format=WEBLINK . Accessed 6 Dec 2021.
Van Eenennaam AL. Method for in vivo x or y sperm selection. Accession no: 1015727. USDA National Institute of Food and Agriculture, Washington, DC. 2018. https://cris.nifa.usda.gov/cgi-bin/starfinder/0?path=fastlink1.txt&id=anon&pass=&search=(AN=1015727)&format=WEBLINK . Accessed 6 Dec 2021.
Van Eenennaam AL, De Figueiredo SF, Trott JF, Zilberman D. Genetic engineering of livestock: the opportunity cost of regulatory delay. Annu Rev Anim Biosci. 2021;9:453–78.
Vincent AL. Generation of zoonotic influenza resistant recombinant pigs via site-directed technology. Accession no: 0437653. USDA National Institute of Food and Agriculture, Washington, DC. 2019. https://cris.nifa.usda.gov/cgi-bin/starfinder/0?path=fastlink1.txt&id=anon&pass=&search=(AN=0437653)&format=WEBLINK . Accessed 6 Dec 2021.
Walker T. Hybrid catfish a huge success. Aquaculture North America. 2015. https://www.aquaculturenorthamerica.com/hybrid-catfish-a-huge-success-1254/ . Accessed 23 Nov 2021.
Wall RJ, Hyman P, Kerr D, Pintado B, Wells K. Transgenic animal technology. J Androl. 1997a;18:236–9.
CAS PubMed Google Scholar
Wall RJ, Kerr DE, Bondioli KR. Transgenic dairy cattle: genetic engineering on a large scale. J Dairy Sci. 1997b;80:2213–24. https://doi.org/10.3168/jds.S0022-0302(97)76170-8 .
Wall RJ, Powell AM, Paape MJ, Kerr DE, Bannerman DD, Pursel VG, Wells KD, Talbot N, Hawk HW. Genetically enhanced cows resist intramammary Staphylococcus aureus infection. Nat Biotechnol. 2005;23:445–51.
Wheeler MB. Transgenic animals in agriculture. Nat Educ Knowl. 2013;4:1.
Google Scholar
Whelan AI, Lema MA. Regulatory framework for gene editing and other new breeding techniques (NBTs) in Argentina. GM Crops Food. 2015;6:253–65. https://doi.org/10.1080/21645698.2015.1114698 .
Whelan AI, Gutti P, Lema MA. Gene editing regulation and innovation economics. Front Bioeng Biotechnol. 2020. https://doi.org/10.3389/fbioe.2020.00303 .
Whitelaw CBA, Sheets TP, Lillico SG, Telugu BP. Engineering large animal models of human disease. J Pathol. 2016;238:247–56. https://doi.org/10.1002/path.4648 ( Epub 2015 Nov 28 ).
Wiggans GB, Cole JB, Hubbard SM, Sonstegard TS. Genomic selection in dairy cattle: the USDA experience. Annu Rev Anim Biosci. 2017;5:309–27. https://doi.org/10.1146/annurev-animal-021815-111422 .
Williams JO. Mule Production. USDA Farmers’ Bulletin No. 1341. 1923. https://naldc.nal.usda.gov/download/5420608/PDF . Accessed 16 Nov 2021.
Download references
Acknowledgements
The authors appreciate input and review of the manuscript provided by Joan Lunney and other USDA colleagues.
This work was supported by the U.S. Department of Agriculture.
Author information
Authors and affiliations.
Office of the Chief Scientist, U.S. Department of Agriculture, 1400 Independence Avenue SW, Washington, DC, 20250, USA
Diane Wray-Cahen
Office of the Chief Economist, U.S. Department of Agriculture, 1400 Independence Avenue SW, Washington, DC, 20250, USA
Anastasia Bodnar
Agricultural Research Service, U.S. Department of Agriculture, 5601 Sunnyside Avenue, Beltsville, MD, 20705, USA
Caird Rexroad III
U.S. Department of Agriculture, National Institute of Food and Agriculture, 805 Pennsylvania Avenue, Kansas City, MO, 64105, USA
Frank Siewerdt
Foreign Agricultural Service, U.S. Department of Agriculture, 1400 Independence Avenue SW, Washington, DC, 20250, USA
You can also search for this author in PubMed Google Scholar
Contributions
All authors contributed to the writing, revisions and made additions to the manuscript. All authors researched the literature. DWC, AB, and CR contributed to the figures. All authors read and approved the final manuscript.
Authors’ information
Diane Wray-Cahen, Senior Advisor for Animal Health and Production, and Animal Products.
Anastasia Bodnar, Agricultural Biotechnology Advisor and USDA Biotechnology Coordinator.
Caird Rexroad III, National Program Leader for Aquaculture.
Frank Siewerdt, National Program Leader for Genetics, Genomics, and Welfare of Agricultural Animals.
Dan Kovich, Science Advisor for New Technologies & Production Methods.
Corresponding author
Correspondence to Diane Wray-Cahen .
Ethics declarations
Ethics approval and consent to participate, consent for publication.
All authors have consented to this submission.
Competing interests
The authors declare that they have no competing interests. Mention of any company or product is for informational purposes and does not represent endorsement by the U.S. Department of Agriculture. The findings and conclusions in this publication are those of the authors and should not be construed to represent any official USDA or U.S. Government determination or policy.
Additional information
Publisher's note.
Springer Nature remains neutral with regard to jurisdictional claims in published maps and institutional affiliations.
Rights and permissions
Open Access This article is licensed under a Creative Commons Attribution 4.0 International License, which permits use, sharing, adaptation, distribution and reproduction in any medium or format, as long as you give appropriate credit to the original author(s) and the source, provide a link to the Creative Commons licence, and indicate if changes were made. The images or other third party material in this article are included in the article's Creative Commons licence, unless indicated otherwise in a credit line to the material. If material is not included in the article's Creative Commons licence and your intended use is not permitted by statutory regulation or exceeds the permitted use, you will need to obtain permission directly from the copyright holder. To view a copy of this licence, visit http://creativecommons.org/licenses/by/4.0/ . The Creative Commons Public Domain Dedication waiver ( http://creativecommons.org/publicdomain/zero/1.0/ ) applies to the data made available in this article, unless otherwise stated in a credit line to the data.
Reprints and permissions
About this article
Cite this article.
Wray-Cahen, D., Bodnar, A., Rexroad, C. et al. Advancing genome editing to improve the sustainability and resiliency of animal agriculture. CABI Agric Biosci 3 , 21 (2022). https://doi.org/10.1186/s43170-022-00091-w
Download citation
Received : 08 March 2022
Accepted : 30 March 2022
Published : 21 April 2022
DOI : https://doi.org/10.1186/s43170-022-00091-w
Share this article
Anyone you share the following link with will be able to read this content:
Sorry, a shareable link is not currently available for this article.
Provided by the Springer Nature SharedIt content-sharing initiative
- Animal breeding
- Public policy
- Regulatory approach
- International trade
- Public acceptance
- Biotechnology
- Aquaculture
CABI Agriculture and Bioscience
ISSN: 2662-4044
- Submission enquiries: Access here and click Contact Us
- General enquiries: [email protected]
Thank you for visiting nature.com. You are using a browser version with limited support for CSS. To obtain the best experience, we recommend you use a more up to date browser (or turn off compatibility mode in Internet Explorer). In the meantime, to ensure continued support, we are displaying the site without styles and JavaScript.
- View all journals
- Explore content
- About the journal
- Publish with us
- Sign up for alerts
- Correspondence
- Published: 25 June 2024
Increasing access to biotech products for animal agriculture in Sub-Saharan Africa through partnerships
- José de la Fuente ORCID: orcid.org/0000-0001-7383-9649 1 ,
- Christian Gortázar 1 ,
- Marinela Contreras 1 ,
- Frederick Kabi ORCID: orcid.org/0000-0002-9868-1603 2 ,
- Paul Kasaija 2 ,
- Swidiq Mugerwa 2 &
- Justus Rutaisire 2
Nature Biotechnology volume 42 , pages 1013–1014 ( 2024 ) Cite this article
269 Accesses
20 Altmetric
Metrics details
- Developing world
- Recombinant vaccine
The biotech products used in developing countries to improve food production and safety are often created and manufactured abroad. Using the example of cattle tick vaccines in Uganda, we highlight the value of collaborations between low-income countries and foreign partners as a means of promoting the development of biotech products for animal agriculture that are tailored to local needs and manufactured in regional facilities.
A related concern is the relevance of new vaccines to regional tick and pathogen species and genotypes. Regional manufacturers of generic products generally lack the capacity to develop new vaccines, and although large multinational companies may have the necessary capacity, they have little incentive to develop products targeted at regional diseases 3 . Additionally, the presence of poor-quality or adulterated drugs remains a major threat to food production and safety in many African countries 2 .
This is a preview of subscription content, access via your institution
Access options
Access Nature and 54 other Nature Portfolio journals
Get Nature+, our best-value online-access subscription
24,99 € / 30 days
cancel any time
Subscribe to this journal
Receive 12 print issues and online access
195,33 € per year
only 16,28 € per issue
Buy this article
- Purchase on SpringerLink
- Instant access to full article PDF
Prices may be subject to local taxes which are calculated during checkout
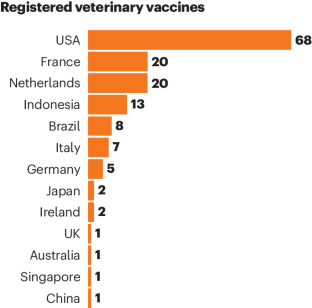
Meeusen, E. N., Walker, J., Peters, A., Pastoret, P. P. & Jungersen, G. Clin. Microbiol. Rev. 20 , 489–510 (2007).
Article CAS PubMed PubMed Central Google Scholar
Grasswitz, T. R. et al. (eds). The veterinary pharmaceutical industry in Africa: a study of Kenya, Uganda and South Africa (African Union/Interafrican Bureau for Animal Resources, 2004); https://fic.tufts.edu/wp-content/uploads/Grasswitz-et-al.pdf
Jaime, G., Hobeika, A. & Figuié, M. Front. Vet. Sci. 8 , 558973 (2022).
Article PubMed PubMed Central Google Scholar
Lubroth, J. et al. Rev. Sci. Tech. 26 , 179–201 (2007).
Article CAS PubMed Google Scholar
de la Fuente, J. et al. Pathogens 12 , 1258 (2023).
Kasaija, P. D., Estrada-Peña, A., Contreras, M., Kirunda, H. & de la Fuente, J. Ticks Tick Borne Dis. 12 , 101756 (2021).
Article PubMed Google Scholar
Kasaija, P. D. et al. Vaccines (Basel) 11 , 99 (2022).
de la Fuente, J. & Rutaisire, J. Curr. Res. Parasitol. Vector Borne Dis. 5 , 100175 (2024).
Kabi, F. et al. Vaccines (Basel) 10 , 1594 (2022).
Download references
Acknowledgements
The authors would like to thank Government of Uganda, National Agricultural Research Organisation, Ministry of Agriculture, Animal Industry and Fisheries, Spanish National Research Council (CSIC), University of Castilla-La Mancha (UCLM), and farmers for their support of this project.
Author information
Authors and affiliations.
Health and Biotechnology (SaBio), Institute for Game and Wildlife Research (IREC, Spanish National Research Council, CSIC – University of Castilla-La Mancha, UCLM – Regional Government of Castile-La Mancha, JCCM), Ciudad Real, Spain
José de la Fuente, Christian Gortázar & Marinela Contreras
National Livestock Resources Research Institute (NaLIRRI), National Agricultural Research Organization (NARO), Kampala, Uganda
Frederick Kabi, Paul Kasaija, Swidiq Mugerwa & Justus Rutaisire
You can also search for this author in PubMed Google Scholar
Contributions
J.F. analyzed data and prepared figures. J.F., C.G., M.C., F.K., S.M. and J.R. conceived the project and organized the consortium. All authors contributed to the content and read and approved the final version of the manuscript.
Corresponding author
Correspondence to José de la Fuente .
Ethics declarations
Competing interests.
The authors declare no competing interests.
Rights and permissions
Reprints and permissions
About this article
Cite this article.
de la Fuente, J., Gortázar, C., Contreras, M. et al. Increasing access to biotech products for animal agriculture in Sub-Saharan Africa through partnerships. Nat Biotechnol 42 , 1013–1014 (2024). https://doi.org/10.1038/s41587-024-02300-5
Download citation
Published : 25 June 2024
Issue Date : July 2024
DOI : https://doi.org/10.1038/s41587-024-02300-5
Share this article
Anyone you share the following link with will be able to read this content:
Sorry, a shareable link is not currently available for this article.
Provided by the Springer Nature SharedIt content-sharing initiative
Quick links
- Explore articles by subject
- Guide to authors
- Editorial policies
Sign up for the Nature Briefing newsletter — what matters in science, free to your inbox daily.


IMAGES
VIDEO
COMMENTS
Atom. RSS Feed. Animal biotechnology is a branch of biotechnology in which molecular biology techniques are used to genetically engineer (i.e. modify the genome of) animals in order to improve ...
Read the latest Research articles in Animal biotechnology from Nature Biotechnology. ... Animal biotechnology articles within Nature Biotechnology. Featured. Article | 10 January 2024.
Animal Biotechnology publishes research on the identification and manipulation of genes and their products, stressing applications in domesticated animals. The journal publishes full-length articles and short research communications, as well as comprehensive reviews. The journal also provides a forum for regulatory or scientific issues related ...
Read the latest Research articles in Animal biotechnology from Nature. ... to improve the governance of research involving animals that contain human genetic or cellular material.
Characterization of chicken Relaxin3 gene: mRNA expression and response to reproductive hormone treatment in ovarian granulosa cells, and single nucleotide polymorphisms associated with egg laying traits in hens. Chunfeng Zhang, Yi Sun, Li Kang & Yunliang Jiang. Published online: 28 Jun 2024. 273 Views.
Understanding biological mechanisms is fundamental for improving animal production and health to meet the growing demand for high-quality protein. As an emerging biotechnology, single-cell transcriptomics has been gradually applied in diverse aspects of animal research, offering an effective method to study the gene expression of high-throughput single cells of different tissues/organs in ...
Animal Biotechnology, Volume 35, Issue 1 (2024) ... Research Article. Article. Sequencing and characterization of complete mitogenome DNA of worldwide turkey (Meleagris gallopavo) populations. Carlotta Ferrari, Stefano P. Marelli, Alessandro Bagnato, Silvia Cerolini & Maria G. Strillacci.
Seema Karanwal, Ankit Pal, Fanny Josan, Aditya Patel, Jatinder Singh Chera, Sonam Yadav, Vikrant Gaur, Preeti Verma, Shiva Badrhan, Vitika Chauhan, Mukesh Bhakat, Tirtha Kumar Datta and Rakesh Kumar. Journal of Animal Science and Biotechnology 2024 15:126. Research Published on: 11 September 2024.
Available online 22 September 2023. Animal biotechnology represents a cutting-edge field that has rev olutionized our interactions with the animal. kingdom. Recent advancements encompass various ...
Animal biotechnology articles within Nature Communications. Featured. Article ... In areas such as animal research and agriculture a single sex is often required in abundance, leading to wasted ...
Journal of Animal Science and Biotechnology is an open access, peer-reviewed journal that encompasses a wide range of research areas including animal genetics, reproduction, nutrition, physiology, biochemistry, biotechnology, feedstuffs and animal products.The journal publishes original and novel research articles and reviews mainly involved in pigs, poultry, beef cattle, cows, goats and sheep ...
Various programs are initiated to investigate the multiple aspects of wildlife bioresources and utilizing them for human and animal welfare and conserving them through management and biotechnological approaches. Box 1. Benefits of Wildlife to Humans. Maintenance of ecological balance through food habits and food chain.
Browse the list of issues and latest articles from Animal Biotechnology. All issues Special issues . Latest articles Open Access; Volume 35 2024 Volume 34 2023 Volume 33 2022 Volume 32 2021 ... Register to receive personalised research and resources by email. Sign me up. Taylor and Francis Group Facebook page. Taylor and Francis Group X Twitter ...
Research ethics committees, animal ethics committees for animal-based research, and institutional research boards for human subjects have a major role in research governance. Some guidelines for the use of experimental or donor animals include assurances of proper conditions for housing animals and minimal pain or discomfort to any animal that ...
Read the latest Research articles in Animal biotechnology from Scientific Reports
Animal agriculture faces unprecedented challenges, including the need to increase productivity to meet increasing demands for high quality protein while combating increasing pest and disease pressures, improving animal welfare, adapting to a changing climate, and reducing the environmental impact of animal agriculture. Genome editing, in concert with other existing technologies, has the ...
The creation of transgenic animals using genetic engineering (GE) technologies is a prominent field of current biotechnology research. The mechanism of introducing an exogenous gene known as a "transgene" (introduced DNA) into a living organism so that the organism will exhibit a new property and transmit that property to its offspring is called transgenesis.
A novel 22-bp InDel within FGF7 gene is significantly associated with growth traits in goat. Xian-feng Wu, Yuan Liu, Ying-gang Wang, Fu Zhang & Wen-yang Li. Published online: 23 Oct 2023. 310 Views. 0 CrossRef citations. 0 Altmetric. Browse the latest articles and research from Animal Biotechnology.
To address these related problems and democratize access to biotech products for animal agriculture, we advocate the establishment of partnerships between research and government units in ...
Animal Biotechnology publishes research on the identification and manipulation of genes and their products, stressing applications in domesticated animals. The journal publishes full-length articles and short research communications, as well as comprehensive reviews. The journal also provides a forum for regulatory or scientific issues related ...
Given animal research is challenged with inadequacies, e.g., animal-to-human knowledge translation, ethical considerations, and cost:benefit, new approach methodologies (NAMs) have been proposed as a replacement. With reference to the field of nutrition and dietetics, our aim was to examine the policies of its leading journals regarding human ...